- 1Alfred-Wegener-Institut Helmholtz-Zentrum für Polar- und Meeresforschung, Biologische Anstalt Helgoland, Helgoland, Germany
- 2FB2, University of Bremen, Bremen, Germany
- 3Reefauna - Spezialisten für Rifftiere, Bremerhaven, Germany
- 4School of Ocean Sciences, College of Environmental Sciences and Engineering, Bangor University, Menai Bridge, United Kingdom
Climate change combined with anthropogenic stressors (e.g. overfishing, habitat destruction) may have particularly strong effects on threatened populations of coastal invertebrates. The collapse of the population of European lobster (Homarus gammarus) around Helgoland constitutes a good example and prompted a large-scale restocking program. The question arises if recruitment of remaining natural individuals and program-released specimens could be stunted by ongoing climate change. We examined the joint effect of ocean warming and acidification on survival, development, morphology, energy metabolism and enzymatic antioxidant activity of the larval stages of the European lobster. Larvae from four independent hatches were reared from stage I to III under a gradient of 10 seawater temperatures (13–24°C) combined with moderate (∼470 µatm) and elevated (∼1160 µatm) seawater pCO2 treatments. Those treatments correspond to the shared socio-economic pathways (SSP), SSP1-2.6 and SSP5-8.5 (i.e. the low and the very high greenhouse gas emissions respectively) projected for 2100 by the Intergovernmental Panel on Climate Change. Larvae under the elevated pCO2 treatment had not only lower survival rates, but also significantly smaller rostrum length. However, temperature was the main driver of energy demands with increased oxygen consumption rates and elemental C:N ratio towards warmer temperatures, with a reducing effect on development time. Using this large temperature gradient, we provide a more precise insight on the aerobic thermal window trade-offs of lobster larvae and whether exposure to the worst hypercapnia scenario may narrow it. This may have repercussions on the recruitment of the remaining natural and program-released specimens and thus, in the enhancement success of future lobster stocks.
Introduction
Since the industrial age, the burning of fossil fuels has led to an exponential increase in CO2 emissions and temperature. To understand the impact of climate change and potential risks, future scenarios have been developed by the Intergovernmental Panel of Climate Change (IPCC), which are based on diverse degrees of mitigation efforts to decrease CO2 emissions. The low mitigation scenario SSP5-8.5, predicts that by the end of this century, sea surface temperature (SST) will have increased by 2.58°C, seawater surface pH will have decreased to 7.7; and atmospheric CO2 could have reached 1000 µatm. In contrast, the high mitigation scenario SSP1-2.6 corresponding mean changes are +0.73°C SST, reduction to pH 8.0 for surface seawater, and approximately 430–480 µatm CO2 concentrations (IPCC, 2014; Pörtner et al., 2019; IPCC, 2021).
The combined effects of ocean acidification (OA) and warming on marine life have been studied for at least two decades, but they are still challenging to interpret and predict. A growing number of experiments using ecologically and economically important species, such as Pacific herring (Villalobos et al., 2020), Pacific oysters (Lemasson et al., 2018), gilthead seabream, meagre (Pimentel et al., 2016), American and European lobster (Small et al., 2015; Waller et al., 2017) have shown an exacerbated impact of OA on survival, physiology, and growth when it was combined with elevated temperatures. Synergistic impacts (the result of stressors interacting and producing a greater effect than the cumulative or individual effects) of climate change vary across life stages with the tendency that early life stages are more sensitive and less tolerant to environmental stressors than adults (Kikkawa et al., 2003; Ishimatsu et al., 2004; Kurihara, 2008). Understanding the synergistic effects of OA and warming on larval development is critical to predict how climate change will influence larval survival, dispersal and hence, population connectivity (Cowen and Sponaugle, 2009; Giménez et al., 2020). This is particularly important for the future of commercially important and vulnerable species, like crustaceans, which have complex life cycles and undergo distinct ontogenetical changes. As in the majority of marine species with planktonic larvae, the transition between the larval pelagic stage to the benthic post-larval stage larvae has been described as a population bottleneck (Marshall and Morgan, 2011).
European lobster (Homarus gammarus) develops through three pelagic larval stages (stages I, II and III), a postlarval stage (stage IV) and then reaches the juvenile stage which marks the complete transition to a benthic lifestyle (Charmantier et al., 1991). The International Union for Conservation of Nature has listed the European lobster as “least concern” as the examination concluded that this species has a broad geographic range, despite commercial fisheries. This is perhaps true on a global level, but not for the lobsters of the German Bight, North Sea, that inhabit the rocky shores of the island of Helgoland. This population experienced a dramatic decline in the 1950s and 1960s from a combination of overfishing, pollution and extensive habitat destruction (Franke and Gutow, 2004). The decline of this population prompted a large-scale restocking program on Helgoland, presently carried out by the lobster conservation company, Reefauna. After 10 years (1999 – 2009) of releasing hatchery-reared juveniles into the wild, the success of the restocking program was evaluated; the results showed that re-stocked lobsters could be re-caught; survival rates averaged 40% and the proportion of caught cultured lobsters to wild lobsters was 3–8% between the years 2007–2009 (Schmalenbach et al., 2011). Nevertheless, despite recapture of marked lobsters, it is unknown whether recruitment is successful. Most of the catches were older individuals and lobster larvae are rarely caught in long-term plankton net monitoring around the island (Greve et al., 2004). Therefore, the question arises if recruitment of remaining natural individuals and program-released specimens is currently successful, or could be affected by ongoing climate change (i.e. ocean warming and acidification).
Only two studies have assessed the joint effects of OA and ocean warming on lobster larvae of the genus Homarus. They provide the first insight on how lobsters may respond to the synergistic effects of environmental changes predicted for the end of the 21st century (Small et al., 2015; Waller et al., 2017). These studies have in common an experimental design based on only two temperatures and two pCO2 regimes, comparing (in a factorial design) ambient temperature and pCO2 conditions with increased temperature and pCO2. Both studies demonstrated that elevated temperature has a stronger effect on life history (survival and development) and physiological responses (oxygen consumption rates) of lobster larvae than elevated pCO2. Nevertheless, it remains unknown how lobsters will react to a broader range of temperatures under ocean acidification. Regional differences from the global mean SST and CO2 uptake trends can result in a “temperature buffering” effect, possibly mitigating some of the negative impacts of OA. Therefore, as suggested by Humphreys (2017), OA experimental setups should be combined with a thermal gradient to reflect regional variation from the global mean SST more realistically.
How marine crustaceans will perform under future high CO2 can be interpreted by their physiological capacities to adjust to environmental change. Crustaceans are water breathers and are directly exposed to ocean acidification through their gills which are specialized for respiratory gas and ion exchange (Taylor and Taylor, 1992). An acute rise in seawater pCO2 reduces (or reverses) the pCO2 diffusion gradient across the gills, causing additional CO2 to accumulate in the haemolymph (extracellular compartment) until an excretory gradient is restored (Whiteley, 2011). Regulating haemolymph pH is necessary to maintain proper oxygen supply, when the concentration of CO2 in the haemolymph increases and pH decreases it causes hemocyanin (oxygen transporting proteins) to release their load of oxygen molecules as explained by the Bohr effect (Hirota et al., 2008; Strobel et al., 2012). Nonetheless, crustaceans are equipped to buffer changes in haemolymph pH to some extent through iono-regulation (Whiteley et al., 1999; Whiteley, 2011). However, acute and long-term exposure to OA could interrupt this acid-base equilibrium and alter metabolism and growth (Whiteley and Taylor, 1992; Whiteley et al., 1999). Thus, routine metabolic rate (RMR) is traditionally investigated in studies on multiple environmental stressors as an approach to assess if elevated CO2 concentrations affect the sensitivity of organisms to thermal extremes (Storch et al., 2011; Waller et al., 2017; Laubenstein et al., 2019). At optimal temperatures, organisms have maximal aerobic capacity and proper functioning (Pörtner, 2001). While at suboptimal temperatures aerobic capacity is limited and failure to sustain a balance between metabolism, development and growth can result in reduced body mass at critical life history stages (Anger, 2001; Pörtner, 2008; Torres and Giménez, 2020). The suboptimal temperatures can be divided into the pejus range, where an organism performance starts to decrease (Frederich and Pörtner, 2000; Frederich et al., 2009); and the pessimus limit, when an organism switches from aerobic into anaerobic metabolism (Jost et al., 2012). Additionally, the energetic costs of maintaining proper functioning under increased pCO2 levels can further interrupt defense mechanisms against reactive oxygen species (ROS), leading to oxidative stress and lipid peroxidation (Rato et al., 2017). Therefore, the decrease in antioxidant response and consequent lipids’ oxidative damage can serve as a proxy to identify when an organism’s defense mechanism has been compromised or suppressed by environmental stressors (Beliaeff and Burgeot, 2002; Rato et al., 2017; Tremblay et al., 2020).
The purpose of our study was to provide a more complete picture on how European lobster larvae will perform in future CO2 conditions by including a wider range of temperature treatments. Our study investigated the ability of European lobster larvae to survive and develop successfully as well as their aerobic metabolic capacity when exposed to the projected SPP1- 2.6 scenario OA conditions and a temperature range that covers cold and warm suboptimal temperatures. Larvae from four independent hatches were reared from stage I to III under a gradient of 10 different seawater temperatures (13–24°C) combined with moderate (average ∼470 µatm) and elevated (average ∼1160 µatm) seawater pCO2 treatments (corresponding to the very stringent [SSP1-2.6] and worst-case emission scenario [SSP5-8.5] projected for 2100 by IPCC).
Materials and Methods
Animal Collection and Maintenance
The study was carried out at AWI Helmholtz-Zentrum für Polar-und Meeresforschung (Helgoland, Germany). The experiment was repeated four times under the same temperature and light regimes (12:12 h light/dark), each experimental run was carried out with larvae from different females or hatches, hereafter referred to hatches. Hatches typically vary due to genetic or maternal effects. Thus, pooling larvae together from different females could potentially mask responses to the treatments. This is the reason why we chose to repeat the experiment with four independent hatches to increase the robustness of the results. European lobster larvae (Homarus gammarus) hatched during summer from four ovigerous female lobsters captured by local fishermen in the rocky subtidal zone around the island of Helgoland (German Bight, North Sea, 54°11:3′N, 7°54.0′E). Females were fed by the lobster conservation company Reefauna and kept in separate tanks (29 × 79 cm), filled with running seawater from the North Sea under a natural light cycle until hatching occurred. Freshly hatched larvae were transferred to 60 × 800 ml glass beakers and were distributed evenly into four sections to minimize cannibalism. Two 100 cm2 plastic meshes (mesh size: 500 µm) were sewn in the middle and placed in the beaker to delimit these areas. The number of larvae was 15 per beaker for the first hatch and was adjusted to 12 afterwards for better survival. In total 2,880 lobster larvae were used. The research presented in this paper complies with the guidelines from the directives 2010/63/EU of the European parliament and of the Council of 22nd September 2010 and the German law on the protection of animals used for scientific purposes.
Experimental Design and Seawater Parameters
A thermal gradient incubator similar to the one used by Thomas et al. (1963) was used for the experimental setup. The table was built to hold 60 beakers (10 columns × 6 rows) and connected to two cooling bath thermostats with engine coolant flowing through a closed system (HUBER Compatible Control K6, Offenburg, Germany) that were set at 11.8 and 27.8°C. A gradient of 10 temperatures was obtained in the horizontal axis (Supplementary Table S1). On the vertical axis, two CO2 concentrations were set at target 450 µatm (SSP1-2.6 scenario) and 1150 µatm (SSP5-8.5 scenario) and supplied with gentle bubbling in three rows for a total of 30 beakers per CO2 concentration. Each beaker had a plastic hose with a glass tube extremity connected to a CO2 distributor. The targeted CO2 levels were reached using a system that removes CO2 from ambient air with a soda lime filter. The CO2-free air (<1 µatm CO2) was mixed with pure CO2 (Air Liquide Deutschland ltd., Düsseldorf, Germany), and the pCO2 of the mixture was continuously monitored with a gas detection unit (GDZ 401, Umsitec, Denkendorf, Germany) that automatically adjusts the CO2 concentration and flow rates to maintain the target values. All beakers were covered by a clear plastic bag to limit CO2 outgassing throughout the experiments. The setup resulted in a triplicate per temperature and CO2 concentration (see Supplementary Table S1). Lobster larvae were raised from stage I to stage III under the temperature and pCO2 conditions related to their position in the gradient table. Larvae were fed ad libitum (ca. 200–300 Artemia salina nauplii) after the daily water change at 9:00.
Seawater parameters were measured daily (n = 1 for each combination of temperature CO2 concentration), using a pH meter (WTW pH315i, Wilheim, Germany) and pH electrode (WTW SenTix 21 Basis pH-combined electrode, Wilheim, Germany), salinometer (WTW Cond 3110 SET 1, Wilheim, Germany) and salinity sensor (WTW, Conductivity Cells TetraCon), and thermometer (VOLTCRAFT DET2R, Wernberg-Köblitz, Germany) (see Supplementary Table S1). Total alkalinity (TA) was measured at the beginning and end of all experimental runs (n = 2 for each combination of temperature CO2 concentration). For TA, water was sampled airtight in 100 ml bottles and stored at 4°C until later measurements with a TitroLine α plus titrator (SI Analytics GmbH [Xylem], Weilheim, Germany) in technical duplicates with Dickson Batch 104 (NOAA, Reference material for oceanic CO2 measurements, 2010) as a standard. The seawater carbonate system was calculated based on measured TA, temperature, pH, salinity and pressure using the CO2SYS Excel Macro software (Pierrot et al., 2006). The following calculations were used, Mehrbach et al. (1973) refitted by Dickson and Millero (1987) for the CO2 constant, total scale (mol/kg-SW) for pH scale, Uppström (1974) for total boron and Dickson (1990) for KHSO4 to calculate the carbonate system. The obtained values are summarized in Supplementary Table S2, the mean values of pCO2 treatments among all temperatures were 467 ± 19 for the moderate pCO2 treatment and 1156 ± 27 for the high pCO2 treatment.
Survival and Development Time
Lobster larvae in each beaker were monitored during the daily water change to record mortality and dead larvae were removed immediately. Cumulative survival was expressed as the percentage of the number of larvae introduced into each beaker at the start of the experimental run. To monitor development, beakers were checked daily at 9:00 for evidence of molting. Larvae were individually observed for stage characteristics, such as the formation of pleopods for stage II and the formation of uropods for stage III. When larvae molted to stage III, they were removed from the beaker for further measurements (see next sections). Sampling was divided into three groups once larvae reached stage III: 1) three larvae from each beaker were sampled for RMR and afterwards frozen for biomass, carbon and nitrogen measurements; 2) three larvae per beaker were photographed for size and morphology analysis and 3) three larvae from each beaker were immediately frozen for enzymatic antioxidants analysis. Each experimental run lasted approximately 28 days, to allow all larvae in different temperatures treatments to reach stage III.
Routine Metabolic Rate (RMR) Measurements
RMR was used as a proxy to investigate the effect of elevated pCO2 and temperature on stage III larvae metabolism. RMR measurements were done under the corresponding experimental temperature and freshly prepared pCO2 conditioned seawater. To make sure larvae were in a post-absorptive state, larvae were starved for 2 h to allow gastric processing (Kurmaly et al., 1990; McGaw and Curtis, 2013) in 20 ml glass vials implemented with an optically isolated oxygen sensor type PSt5 at its bottom (PreSens, Regensburg, Germany). During this 2 h, vials were covered with a mesh to avoid larvae escape and permit oxygen diffusion in the conditioned seawater. This period also allowed larvae to recover from handling stress. After 2 h, vials were tightly closed with a plastic lid, while submerged in the corresponding conditioned seawater in order to avoid air bubbles and placed on a SDR SensorDish® Reader (PreSens, Regensburg, Germany). This system consists of a 24-channel reader of oxygen luminescence quenching and provides a high-quality measurement without oxygen consumption or gas exchange between the environment and the vial functioning as the incubation chamber. The system was calibrated at each temperature with seawater at 100 and 0% air saturation following the manufacturer’s protocol. A 12-well microplate was adapted to the system to measure simultaneously 12 glass vials (20 ml). Vials without larvae (n = 2) were used as a control to account for microbial oxygen consumption. Vials and channel readers were placed on a rocking platform shaker (IKA Rocker 2D digital, Staufen, Germany) at 80 revolutions per minute (rpm) to avoid oxygen stratification within the vials during measurement. The vials were incubated in the dark with an opaque black plastic box. The oxygen concentration was recorded every 15 s during 4 h. Oxygen levels during measurement were monitored closely to avoid suboptimal levels (<4 mg•L−1) inside the chambers. Oxygen consumption was determined by a linear regression of the change in O2 concentration data plotted against time. After RMR was measured larvae were frozen for further biomass, carbon and nitrogen measurements (see next section) to express RMR in O2 mg•h−1•mg DM−1. RMR was measured in postmolt larvae to allow comparison at all temperature treatments, as the intermolt period of larvae is greatly dependent on temperature and is thus highly variable. Past studies measuring RMR in lobster larvae show respiration rates are fairly consistent between intermolt and postmolt stage III larvae (Sasaki et al., 2011).
Biomass, Carbon and Nitrogen Content
Freshly molted stage III larvae used for RMR were sampled for dry body mass, and carbon/nitrogen content measurements. Carbon was measured as a proxy for reserves (lipid content) and nitrogen as a proxy for protein content. The same parameters were measured in freshly hatched larvae (8–15 replicates per hatch) (see Supplementary Table S3). Larvae were rinsed gently with distilled water, blotted dry to remove salts and excess water and stored in 1.5 ml microcentrifuge tubes at −20°C for later analysis. For the analysis, larvae were placed in pre-weighed zinc cartridges (8 × 11 mm, LabNeed, Germany), then freeze-dried for 48 h (Christ Alpha 1–4 freeze dryer, Germany) and afterwards weighed to the nearest 0.0001-mg using a microbalance (Sartorius SC2, Germany). Carbon and nitrogen contents were then measured using an element analyzer (vario MICRO cube CHNS analyzer, Elementar Analysensysteme, Germany).
Dry mass (DM) was measured in freshly hatched larvae (8–15 replicates per hatch) to calculate instantaneous growth. Instantaneous growth rate was calculated as:
In this formula
Morphological Measurements
As a proxy to assess possible malformation under high pCO2, as seen in a study on the effect of OA on lobsters (Agnalt et al., 2013), we measured eight morphological traits. Stage III larvae were placed laterally in a Petri dish and photographed using an Olympus SZX16 stereo microscope. Pictures were then analyzed using ImageJ Software (ImageJ 1.45s, National Institute of Health, Madison, WI, United States). Eight morphological characteristics were measured following the protocol of a similar study in American lobsters (Homarus americanus) (Menu-Courey et al., 2019): 1) rostrum length (RL), 2) carapace length (CL), 3) total length (TL), 4) telson length, 5) the dominant claw pollex, 6) the dactylus, 7) the eye diameter, which consisted of measuring the dark area. The abdomen length was calculated as the difference between TL and the sum of RL and CL.
Antioxidant Enzyme Activity
Stage III larvae were sampled and immediately snap-frozen in liquid N2 and kept at −80°C until analyzed. To determine the level of cellular stress larvae experienced under experimental conditions and the mechanisms involved in the response, four antioxidant enzymes were analyzed in technical triplicates: superoxide dismutase (SOD), glutathione S-transferase (GST), glutathione peroxidase (GPx) and catalase (CAT). Each individual was cut into two pieces below the carapace and ground in liquid N2 using a ceramic pestle. The front part (carapace) was used for antioxidant enzymes. We aimed to quantify lipid damage using the abdomen part of the larva via malondialdehyde (MDA) formation, but these data were discarded as they were mostly under the detection level. For the enzymes’ analysis, the samples were transferred to microcentrifuge tubes with 125 µl of phosphate buffer solution [50 mM potassium phosphate dibasic and monobasic mixture (K2HPO4/KH2PO4, 30.5 and 19.5% respectively), 50 mM Ethylenediaminetetraacetic acid (EDTA), 1 mM phenylmethanesulphonyl fluoride, pH 7.5], homogenised using a laboratory ball mill (MIXER MILL MM 400, Retsch, Haan, Germany) and centrifuged at 23,897 g for 3 min at 4°C to obtain the supernatant used for the assays. SOD catalyses the conversion of O2•− to H2O2 and was measured using xanthine-xanthine oxidase as a superoxide radical generating system and nitroblue tetrazolium as a detector (Suzuki et al., 2000). GST modifies xenobiotics into other conjugates using reduced glutathione (GSH) as substrate, and was estimated by detecting the formation of the thioether product from the reaction between GSH and 1-chloro-2,4-dinitrobenzene (Habig and Jakoby, 1981). GPx removes H2O2 using nicotinamide adenine dinucleotide phosphate (NADPH) as substrate and was measured by monitoring the decrease in the concentration of NADPH at 340 nm upon addition of H2O2 to the assay mixture (Ahmad and Pardini, 1988). CAT eliminates H2O2 too and prevents its accumulation in cells and tissues. The decrease of the H2O2 concentration catalyzed by CAT was measured at 240 nm according to Aebi, (1984). Soluble protein was also measured as per Bradford (1976) in all supernatants to obtain enzyme activities expressed in activity units (U)•mg protein−1. All spectrophotometric measurements were done at room temperature (20°C) using a spectrophotometer (THERMO Multiskan Spectrum, Waltham, United States).
Data Analysis
After data visualization, statistical analyses of the defined variables were performed in RStudio Team (2021). Generalized additive models (GAM) with random effects using the package mgcv (Wood, 2017) were done with temperature and CO2 concentration as fixed factors, plus the addition of the hatch as a random factor (specified as: s(hatch, bs= “re”)) for all measured variables: survival, development time, morphological measurements, RMR, biomass, and antioxidant enzyme activities. The best model (interactive, additive, temperature and CO2 only, null model) was then chosen based on the Akaike information criterion (AIC) score and simplicity of the model (Supplementary Table S4). Line graphs were plotted using the smoothing command from the package mgcv (Wood, 2017) with the predicted regression line in ggplot2 (Wickham, 2016). The lines are the predicted regression lines: solid black lines were plotted when there was a temperature effect, but no effects of OA. Red and blue lines were plotted when there was a temperature and CO2 additive effect; the red and blue dots represent each sampled larva under high or moderate pCO2 conditions respectively. Additionally, a multivariate analysis using a principal component analysis (PCoA) was used to visualize morphological measurements by pCO2 and temperature treatments and permutational multivariate analysis of variance (PERMANOVA) to test significance.
Results
We did not observe any evidence of a synergistic effect of high temperature and high pCO2 in any of the studied variables. Statistically this means that in no case the model including interactions between temperature and pCO2 concentration provided a better fit to the data than the models with the two main factors alone. In general, most variables were affected by temperature and effects of pCO2 (when present) were additive with respect to temperature. We present our results as the average response of larvae under each experimental condition (pCO2 and temperature) and hatch for better visualization. For results separated by hatch see Supplementary Figures S1–S5.
Survival and Development Time
We observed evidence of a negative effect of high pCO2 on larval survival (Supplementary Table S4) but not on development time to reach stage III. At higher temperatures, the differences in average survival between CO2 treatments were small compared to lower temperatures. However, the best model did not retain a term indicating that smooths are conditional on the CO2 level. Overall, mean survival for all temperatures under moderate pCO2 was 33.3% in comparison to 27.9% for high pCO2. Moreover, survival increased with temperature while duration of development time to reach stage III decreased (Figure 1).
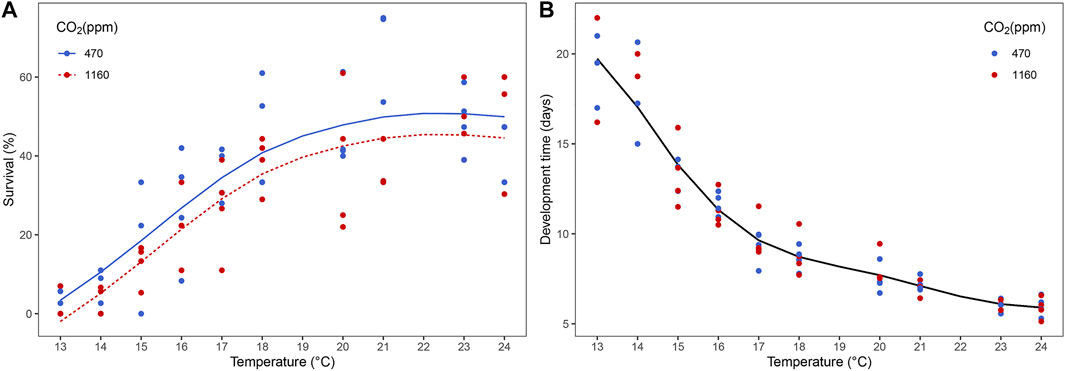
FIGURE 1. Effect of temperature and ocean acidification on the survival and development time of Homarus gammarus larvae to stage III. (A) survival was influenced by CO2 and temperature, (B) development time was influenced by temperature. Each point represents the average response quantified in larvae originated from the same female. Curves correspond to smooths fitted with the best (general additive) model, obtained after backwards model selection.
Biomass and Carbon and Nitrogen Content
Best models retained temperature but not pCO2 as predictors (Figure 2 and Supplementary Table S4). Dry mass, carbon and nitrogen content, and C:N ratio increased with temperature. Instantaneous growth also increased with temperature but there was no evidence of an effect of pCO2 (Supplementary Figure S6). Temperature and pCO2 had an additive effect on total production (Figure 3). This result matches the trend and significance seen in the survival results.
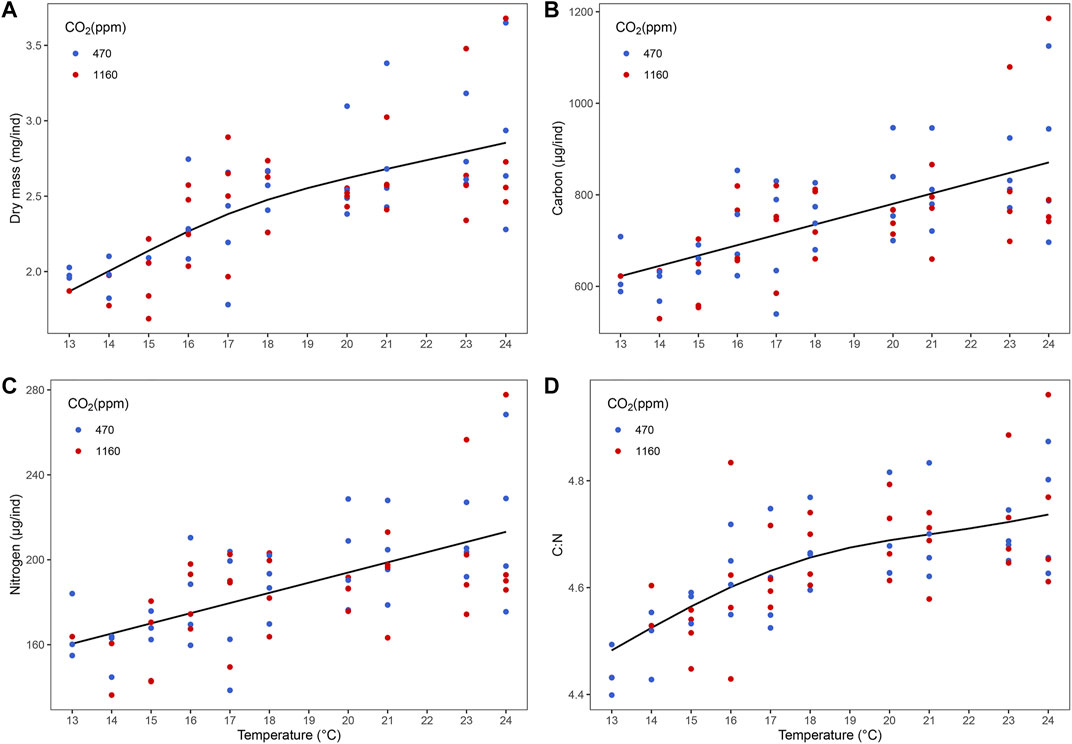
FIGURE 2. Effect of temperature on biomass of stage III Homarus gammarus larvae. (A) dry mass, (B) carbon content, (C) nitrogen content and (D) C:N was positively correlated with temperature. Each point represents the average response quantified in larvae originated from the same female. Curves correspond to smooths fitted with the best (general additive) model, obtained after backwards model selection.
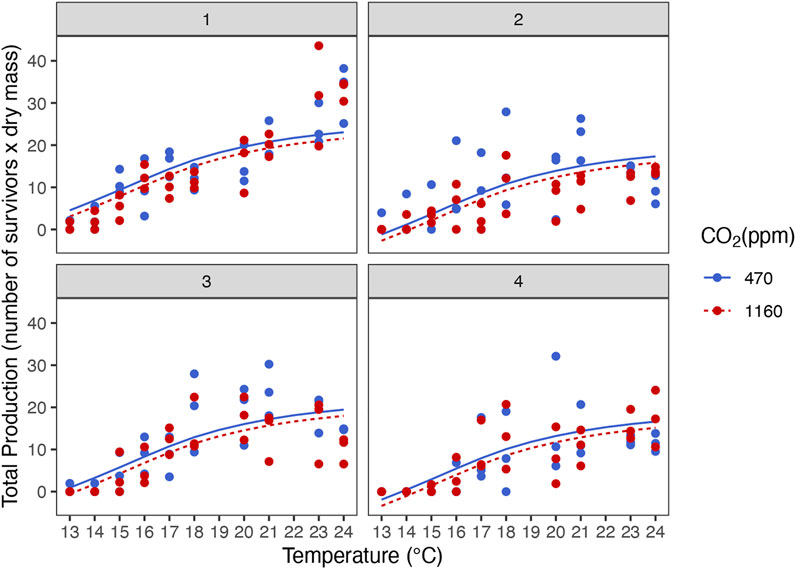
FIGURE 3. Additive effect of temperature and ocean acidification on total production of stage III Homarus gammarus larvae for the four hatches analyzed. Panels 1–4 respectively.
Morphological Measurements
Best models retained both pCO2 and temperature for predictors of rostrum length (Figure 4 and Supplementary Table S4). Lobster larvae in high pCO2 treatment had, on average, shorter rostrum length than those in moderate pCO2. Rostrum length (RL) increased with increasing temperature in both CO2 treatments. For the remaining variables, only temperature was retained in the best model (Supplementary Table S4). Carapace length (CL), abdomen length (AL), total length (TL) and claw size increased with temperature (Figure 4 and Supplementary Table S4). By contrast, the CL:AL ratio decreased with temperature (Figure 4 and Supplementary Table S3). Neither temperature nor pCO2 were retained as predictors for eye diameter size and telson length. Multivariate analysis using measured morphological characteristics (RL, CL, AL, TL and telson) did not give any significant morphological difference between larvae under moderate and high pCO2. Temperature had a significant effect on larval morphology in the colder temperatures (Figure 5; PERMANOVA test: F1, 165 = 7.37, p = 0.003).
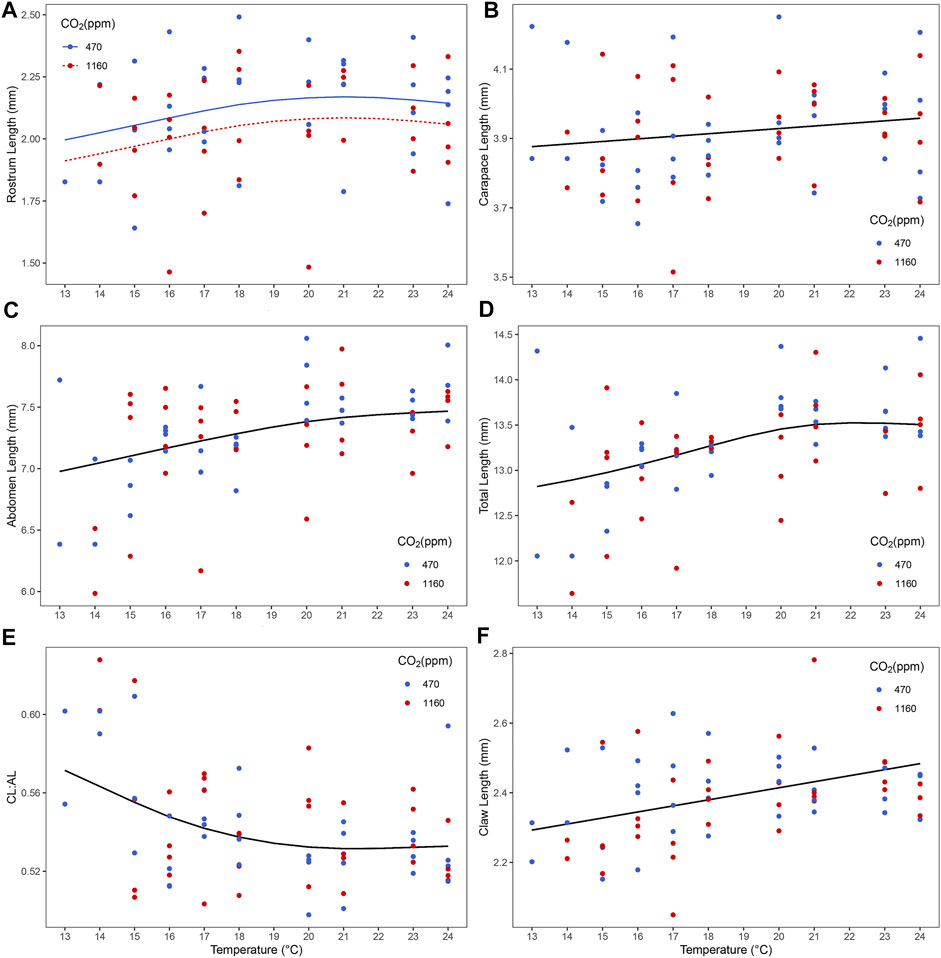
FIGURE 4. Effect of temperature and ocean acidification on size and morphology of stage III Homarus gammarus larvae. (A) ocean acidification and temperature effect on rostrum length; and temperature effect on (B) carapace length [CL], (C) abdomen length [AL], (D) total length, (E) CL: AL ratio and (F) claw length. Each point represents the average response quantified in larvae originated from the same female. Curves correspond to smooths fitted with the best (general additive) model, obtained after backwards model selection.
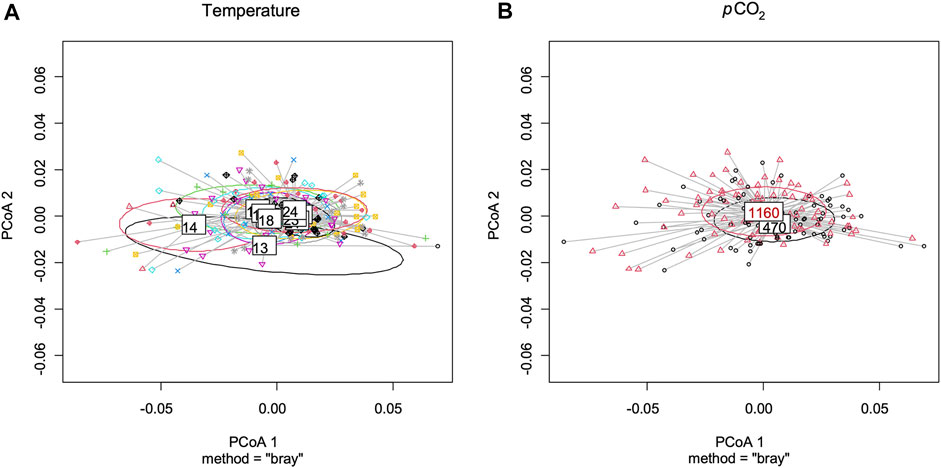
FIGURE 5. Results of principal coordinates analyses (PCoA) for morphological traits of stage III Homarus gammarus larvae. Plots were made using rostrum length, carapace length, abdomen length, total length and telson size. (A) comparison by temperatures (13–24°C), significant differences among temperatures. (PERMANOVA test: F1, 165 = 7.37, p = 0.003). (B) comparison by pCO2 concentrations, no significance differences among pCO2 concentrations.
RMR Measurements and Antioxidant Enzyme Activity
The routine metabolic rate (RMR) increased with temperature (Figure 6 and Supplementary Table S4). However, we did not find any evidence of an effect of OA (i.e. the best model contained only temperature as predictor). Best models did not retain temperature nor CO2 as predictors for variation in antioxidant activity of the enzymes SOD, GST, GPx and CAT (Figure 7).
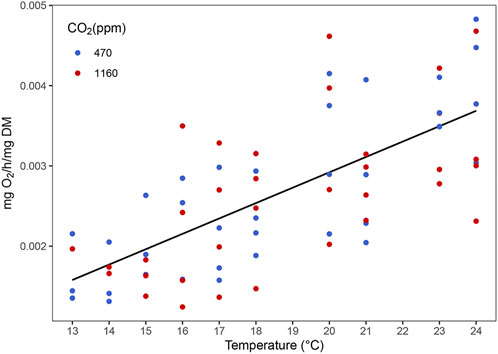
FIGURE 6. Effect of temperature on the routine metabolic rate of stage III Homarus gammarus larvae. Each point represents the average response quantified in larvae originated from the same female. Curves correspond to smooths fitted with the best (general additive) model, obtained after backwards model selection.
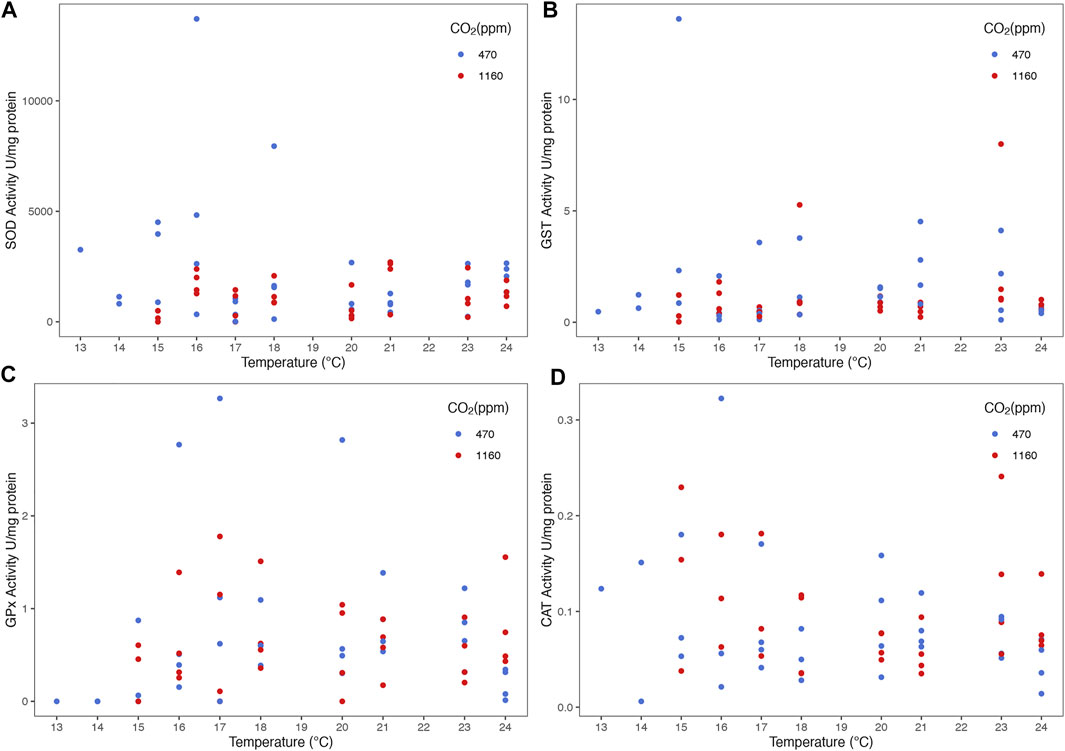
FIGURE 7. Effect of temperature and ocean acidification on antioxidant enzyme activities of stage III Homarus gammarus larvae. There was no effect of experimental conditions (temperature gradient and pCO2 levels) on antioxidant response: (A) SOD, (B) GST, (C) GPx and (D) CAT. Each point represents the average response quantified in larvae originated from the same female. Curves correspond to smooths fitted with the best (general additive) model, obtained after backwards model selection.
Discussion
Contrary to our expectations, we did not find any evidence of synergistic or interactive effects of temperature and pCO2 on any of the studied response variables. Ocean warming and acidification impacts can range from the highest level of sensitivity seen in the whole organism functioning, down to the cellular and molecular levels (Pörtner, 2008). We accordingly discuss our results from whole body functioning (i.e. survival, growth), to energy metabolism and finally to antioxidant responses. Our findings demonstrate that future high CO2 concentrations could have an impact on survival and morphology of lobster larvae. However, at the organizational levels analysed here, we did not detect physiological responses in lobster larvae. The latter will be discussed below.
Survival
Our results suggest that near-future pCO2 conditions have a negative effect on lobster larvae survival to stage III. In lobster larvae, a bottleneck is expected in the transition between the last pelagic stage (stage III) and the benthic (stage IV); the stage III of H. gammarus is the one that accumulates most of the body mass leading to the stage IV (Torres et al., 2021). Such bottlenecks are important as life history transitions (Giménez, 2004; Pechenik, 2006; Marshall and Morgan, 2011). Our study clearly showed OA has the potential to increase mortality before reaching the transitional metamorphic stage (stage III to IV). In the wild, this could translate to an additional obstacle for successful recruitment. Similarly, reduced survival due to elevated pCO2 has been observed in early life stages in the European lobster (Small et al., 2016) and in the congeneric American lobster (Menu-Courey et al., 2019; Noisette et al., 2021). Moreover, this increase in mortality in early life stages of crustaceans exposed to ocean acidification has been noted in several other species: e.g. red king crab, Paralithodes camtschaticus (Long et al., 2013), edible crab, Cancer pagurus (Metzger et al., 2007) and the porcelain crab, Pethrolisthes cinctipes (Ceballos-Osuna et al., 2013). Previous studies on crustaceans and thermal stress revealed elevated pCO2 can narrow the thermal tolerance of the edible crab, C. pagurus and the spider crab, H. araneus (Metzger et al., 2007; Walther et al., 2009; Whiteley, 2011). Our survival results provide no evidence of larvae reaching a temperature threshold or pessimus range on the warm side of our gradient with a maximum temperature (24°C) under high pCO2. However, on the cold side, the low number of lobster larvae that reached stage III at 13 and 14°C in both moderate and high pCO2 treatments suggest the pessimus survival limit is below 15°C. The lack of an interaction shows though that the limits were not affected by OA, in contrast to previous observations (stated above) and Pörtner’s (2008) predictions. The other significant driver for survival was temperature: higher temperatures resulted in higher survival in both moderate and high pCO2 treatments. We observed similarities with a study carried out in the same region (Helgoland) on the effect of climate warming on European lobster larvae (Schmalenbach and Franke 2010). Their results showed that optimal larval survival occurred within 16–22°C which largely matches our results; we also tested warmer temperatures (23°C and 24°C) where survival was even higher.
Growth: Development Time, Biomass, Carbon and Nitrogen Content
There was no evidence of an effect of elevated pCO2 on development time from hatching to stage III. Our results are consistent with studies focusing on temperature only (Schmalenbach and Franke, 2010) as well as pCO2 and temperature (Arnold et al., 2009; Small et al., 2015; Waller et al., 2017) where pCO2 had no effect on lobster larval development rate. This led us to further enquire if there was possibly a trade-off between slower development rate under pCO2. For instance, at moderately low salinities, larval development of H. gammarus is extended, possibly as a way to minimize the negative effects on lipid and protein levels (Torres et al., 2021). Studies on the combined effect of food limitation and increased temperatures have analyzed the integrated response of dry mass and development under different temperatures (Torres and Giménez, 2020; Griffith et al., 2021) to provide insight if delayed development time could be a compensatory response to maintain body mass (and reserves) at stage. We investigated this integrated response, and our results show there was no trade-off between developing slower under elevated pCO2 conditions as larvae were reaching similar biomass when molting to stage III (Figure 8). Temperature alone was the principal driver in development rate, lobster larvae in warmer temperatures molted to stage III faster independently of pCO2 treatment. We did not find any evidence of effects of pCO2 on dry mass and elemental carbon (C) and (N) content, either; thus larvae grew to the thermal-dependent maximum body mass without any need of extending development. If present, the compensatory responses to increased pCO2 levels operated at a different level of organization, potentially at the intracellular level through acid-base balance mechanisms (Whiteley, 2011; Whiteley et al., 2018).
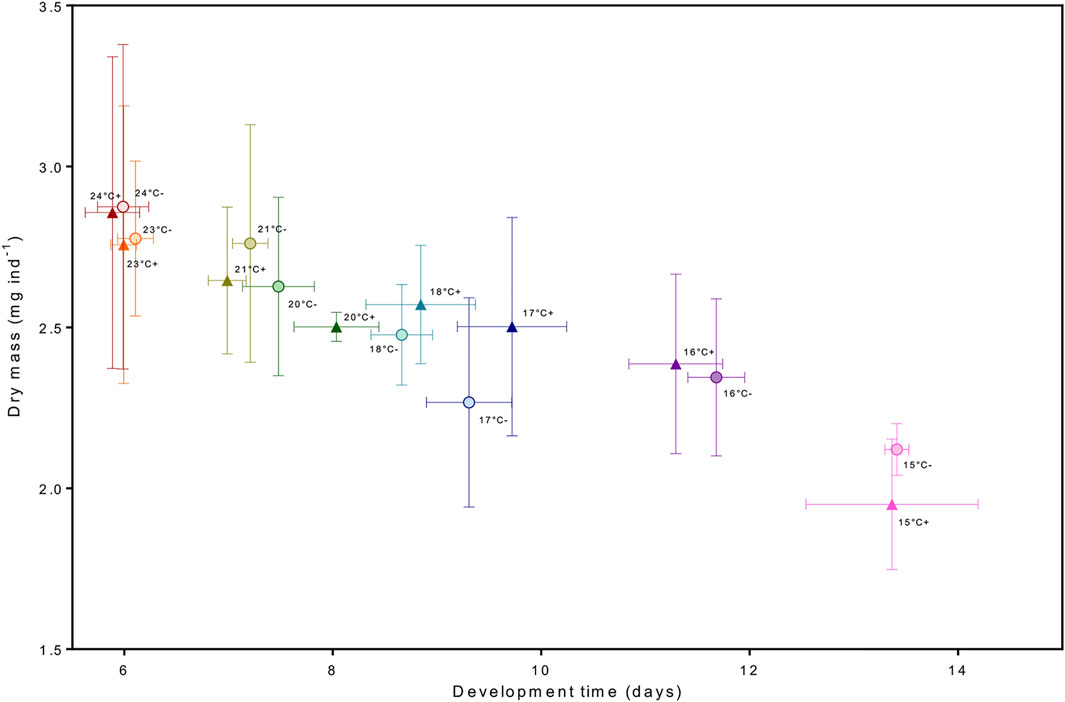
FIGURE 8. Integrated response between development time and drymass. The circle and (−) sign represent the moderate pCO2 treatment and the triangle and (+) sign the high pCO2 treatment.
There was an increase in biomass and C and N content with increasing temperature, consistent with the increased survival. In crustacean larvae, carbon content (approximately >35% of biomass) is correlated with lipid content (a proxy for accumulation of reserves), while nitrogen (approximately 8–11% of biomass) reflects the protein content (Anger and Harms, 1990; Anger, 2001; Torres and Giménez, 2020). Biomass can also be divided into composition of proteins, lipids, chitins and free carbohydrates, making up >30%, <20%, <15%, and <5% respectively (Anger and Harms, 1990; Anger, 1998). However, chitin is mainly associated with cuticle formation and plays a minor role in the accumulation and utilization of energy reserves. Likewise, carbohydrates are stored as glycogen and for the synthesis of non-essential amino acids and thus have no significant influence on the C:N mass quotient (Anger and Harms, 1990).
The effect of temperature on biochemical composition in crustaceans has been studied in both field and controlled laboratory conditions. Field experiments showed that temperature changes, related to seasonal variation, can influence biochemical composition of decapod crustaceans (Buckup et al., 2008; Urzúa and Anger, 2013). Whereas laboratory experiments demonstrated that an increase in temperature led to an augmentation in lipid content in adult male whiteleg shrimps (Litopenaeus vannamei) (Perez-Velazquez et al., 2003) and a decrease in protein content in the northern shrimp larvae (Pandalus borealis) (Brillon et al., 2005). Our results are in line with previous results reporting higher lipid content in cherry shrimp (Neocaridina heteropoda heteropoda) at 24°C (Tropea et al., 2015). However, the same authors noted a decrease in lipid concentrations at 28°C and 32°C, which we did not see with our experimental design. We did not explore extremely high temperature where consumption rates of lipids (reflected in a decrease in carbon content) could have increased due to increased energy demands. More specifically, studies on American and European lobsters have shown varying results that are challenging to compare due to the difference in temperature treatments and life stages. A study done by Small et al. (2016) on juvenile European lobster acclimated at 10°C and 13°C showed carbon content decreased and nitrogen increased in the warmer temperature treatment. Another experiment done on stage I to stage IV European lobster larvae reared at 17°C and 21°C found no significant effects of elevated temperature on organic content (Small et al., 2015). Additionally, an experiment done on OA and warming on American lobster stage III larvae (Waller et al., 2017) showed higher C:N ratio at 19°C compared to 16°C. However, this difference was not seen in stage I, II or IV larvae. Our experiment similarly saw an increase in C:N ratio in stage III larvae at higher temperatures.
The high dry mass, and C and N content matches the energetic demands for rapid growth, accelerated cellular mechanisms and bigger size in warm temperatures. Because C:N ratios were lower towards the lower end of the temperature range, we hypothesize that such low temperatures limited the rate of accumulation of lipids more than that of proteins. Lower changes in proteins than in lipids has also been found when larvae are exposed to low salinity (Torres et al., 2002, 2021). In contrast, on the warmer side an increase in C:N ratio can be indicative of protein degradation due to high metabolism (Weiss et al., 2009). Thus, the decrease seen in C could be related to a degradation of lipids due to extra energetic requirements and a decrease in N could translate to a shortage in protein (Anger and Harms, 1990).
Size and Morphology
Evidence of an effect of high pCO2 on size and morphology was found only for rostrum size, larvae exposed to high pCO2 showing shorter rostrum length than those in the high pCO2 treatment. The rostrum is the region which protects the eyes (Ingle, 1997), a reduced rostrum could potentially lead to eye damage; and proper eye-functioning is necessary for localizing prey and predators (Wahle, 1992). Consequently, we measured the eye diameter, as a proxy to assess if there was any correlation with nervous system underdevelopment (Letourneau, 1976; Laverack, 1988; Beltz and Sandeman, 2003) in elevated pCO2. Nevertheless, despite larvae having smaller rostrum in the high pCO2 there is no evidence that the eye size was influenced by high pCO2 or high temperatures. Additionally, we checked for “puffy” carapace, bent rostrum, and telson deformities, as seen in past studies of juvenile H. gammarus exposed to increased pCO2 at cold (12°C) and optimal temperatures (18°C) (Agnalt et al., 2013). Our results show only one case of a puffy carapace in larva under high pCO2 at 24°C. Lobster larvae reduced size under high pCO2 has been observed before (e.g. in carapace length (Keppel et al., 2012; Rato et al., 2017). Conversely, a study on H. americanus found a positive correlation between elevated pCO2 and carapace and abdomen length (Menu-Courey et al., 2019). We believe the effects on size observed in our study are not as strong as the study by Menu-Courey et al. (2019) and Noisette et al. (2021) possibly because the pCO2 gradient treatments used in those studies reached higher concentrations (1200, 2000 and 3000 µatm) than ours. Furthermore, our study only focused on larval stages and decapod larvae exoskeletons are unmineralized while those of benthic juveniles are partially calcified (Anger, 2001). This lack of calcification may be the reason why elevated pCO2 did not have a stronger impact on the size of the larvae’s different body parts.
RMR and Antioxidant Enzyme Activity
Temperature is one of the most important factors influencing routine metabolic rates (RMR) in lobsters and other decapods (McLeese, 1964). Our results show that lobster larvae RMR increased linearly with increasing temperature, independent of pCO2 treatment. Our findings do not show an exponential relationship between RMR and temperature because this pattern is typically seen in standard and maximal metabolic rate measurements. Moreover, the results are highly dependent on the acclimation of animals, the larvae in our experiments were acclimated to a certain temperature and then respiration rate was measured at the same temperature. Exponential increase of oxygen consumption with temperature is usually seen and obtained with a different methodology (Schulte et al., 2011). A different approach where RMR is measured at acute temperatures independent of the temperature treatment larvae were acclimated to, could have help identify bottlenecks in cell functionality derived from the compensation costs provoked by combined higher temperature and pCO2 treatments. However, the design of our experiment and sample size did not allow for this kind of approach. The “Temperature Induced Metabolic Rate” method could be tested on lobster larvae in the future, as it is suitable for studying the effects of temperature on the metabolic capacities of non-constantly swimming organisms (Paschke et al., 2018). For this standardized method, the researcher is required to evaluate critical thermal maximum (CT max) and critical thermal minimum (CT min) to set the measurement temperature for high and low metabolic rates at each acclimation temperature to calculate an aerobic budget.
The higher RMR at warmer temperatures can be associated with faster development rate and larger size. In physiology, the cost of growing faster comes at the expense of an increase in feeding rates and thus swimming to catch food. These activities have been suggested to be energetically expensive in planktonic crustaceans (Morris et al., 1985). Our results are in line with previous studies on early life stages of Homarus sp. (Small et al., 2015; Waller et al., 2017; Menu-Courey et al., 2019), northern shrimps, Pandalus borealis (Arnberg et al., 2013), and juvenile porcelain crabs, P. cinctipides (Carter et al., 2013), where pCO2 did not significantly affect respiration rates. Significant oxidative stress responses would allow us to infer with more certainty on the optimal, pejus and pessimus ranges of lobster larvae. However, without evidence of significant antioxidant response fluctuations, it is difficult to separate pejus and pessimus ranges. From the oxygen consumption point of view and survival alone, the optimal range would be temperatures between 17–24°C as lobster were able to use their energy supply to maintain maximal physiological functions. Helgoland’s European lobster larvae appear to be quite tolerant to temperatures above those found in the German Bight (Schmalenbach and Franke, 2010).
On the cold side of our temperature gradients, our results show suboptimal temperatures under 15°C, expressed in low survival rates and low RMR. These temperatures are unusual for summertime in Helgoland when lobster larvae hatch (Schmalenbach and Franke, 2010). Nevertheless, temperatures recorded at the Helgoland long-term sampling indicate temperature increases are most noticeable during winter (Franke et al., 1999; Wiltshire et al., 2008). Experimental evidence shows winter warming (+3°C) can alter larval recruitment and result in lobster larvae hatching earlier, mid-April instead of mid-June. In the wild, lobster larvae could be faced with suboptimal temperatures that could cause the lengthening of development time in the pelagic stage, thus increasing the danger of mortality through predation (Schmalenbach and Franke, 2010).
Enzymatic antioxidant responses were measured for the first time in lobster larvae exposed to multiple environmental stressors. To date there is only one study by Rato et al. (2017) that analyzed the biochemical responses of H. gammarus under acidification alone and highlights the occurrence of oxidative stress. They found out lobster larvae under high pCO2 (710 µatm) had reduced SOD and higher DNA damage. Our study included variables not measured before under OA and thermal stress, such as the enzyme activity of GST, GPx and CAT. However, there was no evidence that OA and temperature had a negative impact on the antioxidant enzyme activity. We recognize deeper investigation at the molecular level (proteomic or transcriptomic) could reveal further information on the processes lobster larvae go through to cope with elevated pCO2 (Noisette et al., 2021). For instance, Noisette et al. (2021) findings show elevated pCO2 (up to 3000 µatm) did not have an effect on larvae at a physiological level, however, there is evidence they underwent intensive metabolic reprogramming.
In conclusion, European lobster larvae demonstrated to be resilient to near future pCO2 concentrations at temperatures beyond 17–18°C, including higher temperatures than those experienced by the local population. Our results show larvae do not appear to have reached the critical temperatures or pejus range under the elevated temperatures tested (23–24°C). Raising the temperature even further and reaching the thermal limit of lobster larvae would have been interesting from a physiological point (e.g. higher antioxidant responses and compromised respiration). However, for the purposes of our research question, we wanted to understand how lobster larvae will cope with the predicted SSP5-8.5 scenario for 2100 in which SST will increase by 2-3°C. We observed no interactive effect of temperature and pCO2 on the measured variables; temperature was the greatest driver and there was an additive effect of pCO2 and temperature on survival and size. Examining the results from the perspective of different levels of biological organization, even though pCO2 did not elicit a response at the cellular level (i.e. enzyme activity) or physiological level (i.e development time to reach stage III); at the population level (survival) there were significant negative effects. We used total production (survival times biomass) as a way to integrate physiological and population responses, and it was evident there was an increase in mortality in larvae exposed to high pCO2 accompanied with lower biomass in the suboptimal temperatures (<15°C). Integrating physiological responses to environmental stressors and life history traits is key for species conservation strategies and stock enhancement management. Worst-case climate change scenarios could thus potentially have repercussion on ongoing restock efforts of endangered populations under recovery, like the European lobster population of Helgoland.
Data Availability Statement
The raw data supporting the conclusions of this article will be made available by the authors, without undue reservation.
Author Contributions
MB acquired funding for the experiment. RK provided resources. All authors were involved in experiment design, LL and NT carried out the experiment. LL, NT, and LG analyzed the data. NT, MB, GT, and LG supervised the project. LL wrote the first draft of the manuscript; all authors revised the manuscript.
Funding
This work was funded by the Business Development and Technology Transfer Corporation of Schleswig Holstein (WT.SH) as part of L.L. doctoral thesis.
Conflict of Interest
The authors declare that the research was conducted in the absence of any commercial or financial relationships that could be construed as a potential conflict of interest.
Publisher’s Note
All claims expressed in this article are solely those of the authors and do not necessarily represent those of their affiliated organizations, or those of the publisher, the editors and the reviewers. Any product that may be evaluated in this article, or claim that may be made by its manufacturer, is not guaranteed or endorsed by the publisher.
Acknowledgments
We thank Lorenz Eckardt for his help and technical support during the experimental phase and Julia Haafke for measuring carbon and nitrogen content. The authors would also like to thank Reefauna and Isabel Schmalenbach for providing lobster larvae. We acknowledge support by the Open Access Publication Funds of Alfred-Wegener-Institut Helmholtz-Zentrum für Polar-und Meeresforschung.
Supplementary Material
The Supplementary Material for this article can be found online at: https://www.frontiersin.org/articles/10.3389/fphys.2022.809929/full#supplementary-material
References
Aebi H. (1984). “[13] Catalase In Vitro,” in Methods in Enzymology(Academic Press), 121–126. Oxygen Radicals in Biological Systems. doi:10.1016/S0076-6879(84)05016-3
Agnalt A.-L., Grefsrud E. S., Farestveit E., Larsen M., Keulder F. (2013). Deformities in Larvae and Juvenile European Lobster (Homarus gammarus) Exposed to Lower pH at Two Different Temperatures. Biogeosciences 10, 7883–7895. doi:10.5194/bg-10-7883-2013
Ahmad S., Pardini R. S. (1988). Evidence for the Presence of Glutathione Peroxidase Activity toward an Organic Hydroperoxide in Larvae of the Cabbage Looper Moth, Trichoplusia Ni. Insect Biochem. 18, 861–866. doi:10.1016/0020-1790(88)90111-4
Anger K., Harms J. (1990). Elemental (CHN) and Proximate Biochemical Composition and Decapod Crustacean Larvae. Comp. Biochem. Physiol. Part B Comp. Biochem. 97, 69–80. doi:10.1016/0305-0491(90)90180-2
Anger K. (1998). Patterns of Growth and Chemical Composition in Decapod Crustacean Larvae. Invertebr. Reproduct. Dev. 33, 159–176. doi:10.1080/07924259.1998.9652629
Arnberg M., Calosi P., Spicer J. I., Tandberg A. H. S., Nilsen M., Westerlund S., et al. (2013). Elevated Temperature Elicits Greater Effects Than Decreased pH on the Development, Feeding and Metabolism of Northern Shrimp (Pandalus borealis) Larvae. Mar. Biol. 160, 2037–2048. doi:10.1007/s00227-012-2072-9
Arnold K. E., Findlay H. S., Spicer J. I., Daniels C. L., Boothroyd D. (2009). Effect of CO2 Related Acidification on Aspects of the Larval Development of the European Lobster, Homarus gammarus. Biogeosciences 6, 1747–1754. doi:10.5194/bg-6-1747-2009
Beliaeff B., Burgeot T. (2002). Integrated Biomarker Response: A Useful Tool for Ecological Risk Assessment. Environ. Toxicol. Chem. 21, 1316–1322. doi:10.1002/etc.5620210629
Beltz B. S., Sandeman D. C. (2003). Regulation of Life-Long Neurogenesis in the Decapod Crustacean Brain. Arthropod. Struct. Dev. 32, 39–60. doi:10.1016/S1467-8039(03)00038-0
Bradford M. M. (1976). A Rapid and Sensitive Method for the Quantitation of Microgram Quantities of Protein Utilizing the Principle of Protein-Dye Binding. Anal. Biochem. 72, 248–254. doi:10.1016/0003-2697(76)90527-3
Brillon S., Lambert Y., Dodson J. (2005). Egg Survival, Embryonic Development, and Larval Characteristics of Northern Shrimp (Pandalus borealis) Females Subject to Different Temperature and Feeding Conditions. Mar. Biol. 147, 895–911. doi:10.1007/s00227-005-1633-6
Buckup L., Dutra B. K., Ribarcki F. P., Fernandes F. A., Noro C. K., Oliveira G. T., et al. (2008). Seasonal Variations in the Biochemical Composition of the Crayfish Parastacus defossus (Crustacea, Decapoda) in its Natural Environment. Comp. Biochem. Physiol. Part A Mol. Integr. Physiol. 149, 59–67. doi:10.1016/j.cbpa.2007.10.008
Carter H. A., Ceballos-Osuna L., Miller N. A., Stillman J. H. (2013). Impact of Ocean Acidification on Metabolism and Energetics during Early Life Stages of the Intertidal Porcelain Crab Petrolisthes cinctipes. J. Exp. Biol. 216, 1412–1422. doi:10.1242/jeb.078162
Ceballos-Osuna L., Carter H. A., Miller N. A., Stillman J. H. (2013). Effects of Ocean Acidification on Early Life-History Stages of the Intertidal Porcelain Crab Petrolisthes cinctipes. J. Exp. Biol. 216, 1405–1411. doi:10.1242/jeb.078154
Charmantier G., Charmantier-Daures M., Aiken D. E. (1991). Metamorphosis in the Lobster Homarus (Decapoda): a Review. J. Crustac. Biol. 11, 481–495. doi:10.2307/1548517
Cowen R. K., Sponaugle S. (2009). Larval Dispersal and Marine Population Connectivity. Annu. Rev. Mar. Sci. 1, 443–466. doi:10.1146/annurev.marine.010908.163757
Dickson A. G., Millero F. J. (1987). A Comparison of the Equilibrium Constants for the Dissociation of Carbonic Acid in Seawater Media. Deep Sea Res. Part A. Oceanogr. Res. Pap. 34, 1733–1743. doi:10.1016/0198-0149(87)90021-5
Dickson A. G. (1990). Thermodynamics of the Dissociation of Boric Acid in Synthetic Seawater from 273.15 to 318.15 K. Deep Sea Res. Part A. Oceanogr. Res. Pap. 37, 755–766. doi:10.1016/0198-0149(90)90004-F
Franke H.-D., Gutow L. (2004). Long-term Changes in the Macrozoobenthos Around the Rocky Island of Helgoland (German Bight, North Sea). Helgol. Mar. Res. 58, 303–310. doi:10.1007/s10152-004-0193-3
Franke H.-D., Gutow L., Janke M. (1999). The Recent Arrival of the Oceanic Isopod Idotea metallica Bosc off Helgoland (German Bight, North Sea): an Indication of a Warming Trend in the North Sea? Helgol. Meeresunters. 52, 347–357. doi:10.1007/BF02908908
Frederich M., Pörtner H. O. (2000). Oxygen Limitation of Thermal Tolerance Defined by Cardiac and Ventilatory Performance in Spider Crab, Maja squinado. Am. J. Physiol.-Regul. Integr. Comp. Physiol. 279, R1531–R1538. doi:10.1152/ajpregu.2000.279.5.R1531
Frederich M., O'Rourke M. R., Furey N. B., Jost J. A. (2009). AMP-Activated Protein Kinase (AMPK) in the Rock Crab, Cancer irroratus : an Early Indicator of Temperature Stress. J. Exp. Biol. 212, 722–730. doi:10.1242/jeb.021998
Giménez L., Robins P., Jenkins S. R. (2020). Role of Trait Combinations, Habitat Matrix, and Network Topology in Metapopulation Recovery from Regional Extinction. Limnol. Oceanogr. 65, 775–789. doi:10.1002/lno.11347
Giménez L. (2004). Marine Community Ecology: Importance of Trait-Mediated Effects Propagating through Complex Life Cycles. Mar. Ecol. Prog. Ser. 283, 303–310. doi:10.3354/meps283303
Greve W., Reiners F., Nast J., Hoffmann S. (2004). Helgoland Roads Meso- and Macrozooplankton Time-Series 1974 to 2004: Lessons from 30 Years of Single Spot, High Frequency Sampling at the Only Off-Shore Island of the North Sea. Helgol. Mar. Res. 58, 274–288. doi:10.1007/s10152-004-0191-5
Griffith K., Jenkins S. R., Giménez L. (2021). Larval Tolerance to Food Limitation Is Stronger in an Exotic Barnacle Than in its Native Competitor. Zoology 145, 125891. doi:10.1016/j.zool.2020.125891
Habig W. H., Jakoby W. B. (1981). Assays for Differentiation of Glutathione S-Transferases. Methods Enzymol. 77, 398–405. doi:10.1016/S0076-6879(81)77053-8
Hirota S., Kawahara T., Beltramini M., Di Muro P., Magliozzo R. S., Peisach J., et al. (2008). Molecular Basis of the Bohr Effect in Arthropod Hemocyanin. J. Biol. Chem. 283, 31941–31948. doi:10.1074/jbc.M803433200
Humphreys M. P. (2017). Climate Sensitivity and the Rate of Ocean Acidification: Future Impacts, and Implications for Experimental Design. ICES J. Mar. Sci. 74, 934–940. doi:10.1093/icesjms/fsw189
Ingle R. (1997). “Structure and Function,” in Crayfishes, Lobsters and Crabs of Europe: An Illustrated Guide to Common and Traded Species. Editor R. Ingle (Dordrecht: Springer Netherlands), 1–10. doi:10.1007/978-94-011-5872-5_1
IPCC (2014). in Climate Change 2014: Synthesis Report. Contribution of Working Groups I, II and III to the Fifth Assessment Report of the Intergovernmental Panel on Climate Change. Editors R. K. Pachauri, and L. A. Meyer (Geneva, Switzerland: IPCC), 151.
IPCC (2021). Climate Change 2021: The Physical Science Basis. Contribution of Working Group I to the Sixth Assessment Report of the Intergovernmental Panel on Climate Change. Cambridge, United Kingdom and New York, NY: Cambridge University Press.
Ishimatsu A., Kikkawa T., Hayashi M., Lee K.-S., Kita J. (2004). Effects of CO2 on Marine Fish: Larvae and Adults. J. Oceanogr. 60, 731–741. doi:10.1007/s10872-004-5765-y
Jost J., Podolski S., Frederich M. (2012). Enhancing Thermal Tolerance by Eliminating the Pejus Range: a Comparative Study with Three Decapod Crustaceans. Mar. Ecol. Prog. Ser. 444, 263–274. doi:10.3354/meps09379
Keppel E. A., Scrosati R. A., Courtenay S. C. (2012). Ocean Acidification Decreases Growth and Development in American Lobster (Homarus americanus ) Larvae. J. N. Atl. Fish. Sci. 44, 61–66. doi:10.2960/J.v44.m683
Kikkawa T., Ishimatsu A., Kita J. (2003). Acute CO2 Tolerance during the Early Developmental Stages of Four Marine Teleosts. Environ. Toxicol. 18, 375–382. doi:10.1002/tox.10139
Kurihara H. (2008). Effects of CO2-driven Ocean Acidification on the Early Developmental Stages of Invertebrates. Mar. Ecol. Prog. Ser. 373, 275–284. doi:10.3354/meps07802
Kurmaly K., Jones D. A., Yule A. B. (1990). Acceptability and Digestion of Diets Fed to Larval Stages of Homarus gammarus and the Role of Dietary Conditioning Behaviour. Mar. Biol. 106, 181–190. doi:10.1007/BF01314799
Laubenstein T. D., Rummer J. L., McCormick M. I., Munday P. L. (2019). A Negative Correlation between Behavioural and Physiological Performance under Ocean Acidification and Warming. Sci. Rep. 9, 4265. doi:10.1038/s41598-018-36747-9
Laverack M. S. (1988). The Numbers of Neurones in Decapod Crustacea. J. Crustacean Biol. 8, 1–11. doi:10.2307/1548424
Lemasson A. J., Hall-Spencer J. M., Fletcher S., Provstgaard-Morys S., Knights A. M. (2018). Indications of Future Performance of Native and Non-native Adult Oysters under Acidification and Warming. Mar. Environ. Res. 142, 178–189. doi:10.1016/j.marenvres.2018.10.003
Letourneau J. G. (1976). Addition of Sensory Structures and Associated Neurons to the Crayfish Telson during Development. J. Comp. Physiol. 110, 13–23. doi:10.1007/BF00656778
Long W. C., Swiney K. M., Harris C., Page H. N., Foy R. J. (2013). Effects of Ocean Acidification on Juvenile Red King Crab (Paralithodes camtschaticus) and Tanner Crab (Chionoecetes bairdi) Growth, Condition, Calcification, and Survival. PLOS ONE 8, e60959. doi:10.1371/journal.pone.0060959
Marshall D. J., Morgan S. G. (2011). Ecological and Evolutionary Consequences of Linked Life-History Stages in the Sea. Curr. Biol. 21, R718–R725. doi:10.1016/j.cub.2011.08.022
McGaw I. J., Curtis D. L. (2013). A Review of Gastric Processing in Decapod Crustaceans. J. Comp. Physiol. B 183, 443–465. doi:10.1007/s00360-012-0730-3
McLeese D. W. (1964). Oxygen Consumption of the Lobster, Homarus americanus Milne-Edwards. Helgol. Wiss. Meeresunters. 10, 7–18. doi:10.1007/BF01626094
Mehrbach C., Culberson C. H., Hawley J. E., Pytkowicx R. M. (1973). Measurement of the Apparent Dissociation Constants of Carbonic Acid in Seawater at Atmospheric Pressure1. Limnol. Oceanogr. 18, 897–907. doi:10.4319/lo.1973.18.6.0897
Menu-Courey K., Noisette F., Piedalue S., Daoud D., Blair T., Blier P. U., et al. (2019). Energy Metabolism and Survival of the Juvenile Recruits of the American Lobster (Homarus americanus) Exposed to a Gradient of Elevated Seawater pCO2. Mar. Environ. Res. 143, 111–123. doi:10.1016/j.marenvres.2018.10.002
Metzger R., Sartoris F. J., Langenbuch M., Pörtner H. O. (2007). Influence of Elevated CO2 Concentrations on Thermal Tolerance of the Edible Crab Cancer pagurus. J. Therm. Biol. 32, 144–151. doi:10.1016/j.jtherbio.2007.01.010
Morris M. J., Gust G., Torres J. J. (1985). Propulsion Efficiency and Cost of Transport for Copepods: a Hydromechanical Model of Crustacean Swimming. Mar. Biol. 86, 283–295. doi:10.1007/BF00397515
Noisette F., Calosi P., Madeira D., Chemel M., Menu-Courey K., Piedalue S., et al. (2021). Tolerant Larvae and Sensitive Juveniles: Integrating Metabolomics and Whole-Organism Responses to Define Life-Stage Specific Sensitivity to Ocean Acidification in the American Lobster. Metabolites 11, 584. doi:10.3390/metabo11090584
Paschke K., Agüero J., Gebauer P., Díaz F., Mascaró M., López-Ripoll E., et al. (2018). Comparison of Aerobic Scope for Metabolic Activity in Aquatic Ectotherms with Temperature Related Metabolic Stimulation: A Novel Approach for Aerobic Power Budget. Front. Physiol. 9, 1438. doi:10.3389/fphys.2018.01438
Pechenik J. A. (2006). Larval Experience and Latent Effects—Metamorphosis Is Not a New Beginning. Integr. Comp. Biol. 46, 323–333. doi:10.1093/icb/icj028
Perez-Velazquez M., González-Félix M. L., Lawrence A. L., Gatlin D. M. (2003). Changes in Lipid Class and Fatty Acid Composition of Adult Male Litopenaeus vannamei (Boone) in Response to Culture Temperature and Food Deprivation. Aquac. Res. 34, 1205–1213. doi:10.1046/j.1365-2109.2003.00931.x
Pierrot D., Lewis E., Wallace D. W. R. (2006). MS Excel Program Developed for CO2 System Calculations. Oak Ridge, Tennessee: Carbon Dioxide Information Analysis Center, Oak Ridge National Laboratory, U.S. Department of Energy. ORNL/CDIAC-105a. doi:10.3334/CDIAC/otg.CO2SYS_XLS_CDIAC105a
Pimentel M. S., Faleiro F., Marques T., Bispo R., Dionísio G., Faria A. M., et al. (2016). Foraging Behaviour, Swimming Performance and Malformations of Early Stages of Commercially Important Fishes under Ocean Acidification and Warming. Clim. Change 137, 495–509. doi:10.1007/s10584-016-1682-5
Pörtner H. O., Roberts D. C., Masson-Delmotte V., Zhai P., Tignor M., Poloczanska E., et al. (2019). IPCC Special Report on the Ocean and Cryosphere in a Changing Climate. IPCC Intergov. Panel Clim. Change Geneva Switz. 1, 755. doi:10.1017/9781009157964
Pörtner H. O. (2001). Climate Change and Temperature-dependent Biogeography: Oxygen Limitation of Thermal Tolerance in Animals. Naturwissenschaften 88, 137–146. doi:10.1007/s001140100216
Pörtner H. (2008). Ecosystem Effects of Ocean Acidification in Times of Ocean Warming: a Physiologist's View. Mar. Ecol. Prog. Ser. 373, 203–217. doi:10.3354/meps07768
Rato L. D., Novais S. C., Lemos M. F. L., Alves L. M. F., Leandro S. M. (2017). Homarus gammarus (Crustacea: Decapoda) Larvae under an Ocean Acidification Scenario: Responses across Different Levels of Biological Organization. Comp. Biochem. Physiol. Part C Toxicol. Pharmacol. 203, 29–38. doi:10.1016/j.cbpc.2017.09.002
RStudio Team (2021). RStudio: Integrated Development for R. Boston, MA: RStudio. Available at: http://www.rstudio.com/.
Sasaki G. C., Capuzzo J. M., Biesiot P. (2011). Nutritional and Bioenergetic Considerations in the Development of the American Lobster Homarus americanus. Can. J. Fish. Aquat. Sci. 43, 2311–2319. doi:10.1139/f86-283
Schmalenbach I., Franke H.-D. (2010). Potential Impact of Climate Warming on the Recruitment of an Economically and Ecologically Important Species, the European Lobster (Homarus gammarus) at Helgoland, North Sea. Mar. Biol. 157, 1127–1135. doi:10.1007/s00227-010-1394-8
Schmalenbach I., Mehrtens F., Janke M., Buchholz F. (2011). A Mark-Recapture Study of Hatchery-Reared Juvenile European Lobsters, Homarus gammarus, Released at the Rocky Island of Helgoland (German Bight, North Sea) from 2000 to 2009. Fish. Res. 108, 22–30. doi:10.1016/j.fishres.2010.11.016
Schulte P. M., Healy T. M., Fangue N. A. (2011). Thermal Performance Curves, Phenotypic Plasticity, and the Time Scales of Temperature Exposure. Integr. Comp. Biol. 51, 691–702. doi:10.1093/icb/icr097
Small D. P., Calosi P., Boothroyd D., Widdicombe S., Spicer J. I. (2015). Stage-Specific Changes in Physiological and Life-History Responses to Elevated Temperature and pCO2 during the Larval Development of the European Lobster Homarus gammarus (L.). Physiol. Biochem. Zool. 88, 494–507. doi:10.1086/682238
Small D. P., Calosi P., Boothroyd D., Widdicombe S., Spicer J. I. (2016). The Sensitivity of the Early Benthic Juvenile Stage of the European Lobster Homarus gammarus (L.) to Elevated pCO2 and Temperature. Mar. Biol. 163, 53. doi:10.1007/s00227-016-2834-x
Storch D., Fernández M., Navarrete S., Pörtner H. (2011). Thermal Tolerance of Larval Stages of the Chilean Kelp Crab Taliepus dentatus. Mar. Ecol. Prog. Ser. 429, 157–167. doi:10.3354/meps09059
Strobel A., Hu M. Y. A., Gutowska M. A., Lieb B., Lucassen M., Melzner F., et al. (2012). Influence of Temperature, Hypercapnia, and Development on the Relative Expression of Different Hemocyanin Isoforms in the Common Cuttlefish Sepia officinalis: Differential Hemocyanin Expression in Cuttlefish. J. Exp. Zool. 317, 511–523. doi:10.1002/jez.1743
Suzuki M., Takeuchi H., Kakita T., Unno M., Katayose Y., Matsuno S. (2000). The Involvement of the Intracellular Superoxide Production System in Hepatic Ischemia-Reperfusion Injury: In Vivo and In Vitro Experiments Using Transgenic Mice Manifesting Excessive CuZn-SOD Activity. Free Radic. Biol. Med. 29, 756–763. doi:10.1016/S0891-5849(00)00369-5
Taylor E., Taylor H. (1992). Gills and Lungs : The Exchange of Gases and Ions. Microsc. Anat. Invertebr. 10, 203–293. Available at: https://ci.nii.ac.jp/naid/10020942339/ (Accessed November 1, 2021).
Thomas W. H., Scotten H. L., Bradshaw J. S. (1963). Thermal Gradient Incubators for Small Aquatic Organisms1. Limnol. Oceanogr. 8, 357–360. doi:10.4319/lo.1963.8.3.0357
Torres G., Giménez L. (2020). Temperature Modulates Compensatory Responses to Food Limitation at Metamorphosis in a Marine Invertebrate. Funct. Ecol. 34, 1564–1576. doi:10.1111/1365-2435.13607
Torres G., Giménez L., Anger K. (2002). Effects of Reduced Salinity on the Biochemical Composition (Lipid, Protein) of Zoea 1 Decapod Crustacean Larvae. J. Exp. Mar. Biol. Ecol. 277, 43–60. doi:10.1016/S0022-0981(02)00244-7
Torres G., Anger K., Giménez L. (2021). Effects of Short-Term and Continuous Exposure to Reduced Salinities on the Biochemical Composition of Larval Lobster, Homarus gammarus. Zoology 144, 125885. doi:10.1016/j.zool.2020.125885
Tremblay N., Guerra-Castro E. J., Díaz F., Rodríguez-Fuentes G., Simões N., Robertson D. R., et al. (2020). Cold Temperature Tolerance of the Alien Indo-Pacific Damselfish Neopomacentrus cyanomos from the Southern Gulf of Mexico. J. Exp. Mar. Biol. Ecol. 524, 151308. doi:10.1016/j.jembe.2019.151308
Tropea C., Stumpf L., López Greco L. S. (2015). Effect of Temperature on Biochemical Composition, Growth and Reproduction of the Ornamental Red Cherry Shrimp Neocaridina heteropoda heteropoda (Decapoda, Caridea). PLOS ONE 10, e0119468. doi:10.1371/journal.pone.0119468
Uppström L. R. (1974). The Boron/chlorinity Ratio of Deep-Sea Water from the Pacific Ocean. Deep Sea Res. Oceanogr. Abstr. 21, 161–162. doi:10.1016/0011-7471(74)90074-6
Urzúa Á., Anger K. (2013). Seasonal Variations in Larval Biomass and Biochemical Composition of Brown Shrimp, Crangon crangon (Decapoda, Caridea), at Hatching. Helgol. Mar. Res. 67, 267–277. doi:10.1007/s10152-012-0321-4
Villalobos C., Love B. A., Olson M. B. (2020). Ocean Acidification and Ocean Warming Effects on Pacific Herring (Clupea pallasi) Early Life Stages. Front. Mar. Sci. 7, 597899. doi:10.3389/fmars.2020.597899
Wahle R. A. (1992). Body-Size Dependent Anti-predator Mechanisms of the American Lobster. Oikos 65, 52–60. doi:10.2307/3544887
Waller J. D., Wahle R. A., McVeigh H., Fields D. M. (2017). Linking Rising pCO2 and Temperature to the Larval Development and Physiology of the American Lobster (Homarus americanus). ICES J. Mar. Sci. 74, 1210–1219. doi:10.1093/icesjms/fsw154
Walther K., Sartoris F. J., Bock C., Pörtner H. O. (2009). Impact of Anthropogenic Ocean Acidification on Thermal Tolerance of the Spider Crab Hyas araneus. Biogeosciences 6, 2207–2215. doi:10.5194/bg-6-2207-2009
Weiss M., Heilmayer O., Brey T., Thatje S. (2009). Influence of Temperature on the Zoeal Development and Elemental Composition of the Cancrid Crab, Cancer setosus Molina, 1782 from Pacific South America. J. Exp. Mar. Biol. Ecol. 376, 48–54. doi:10.1016/j.jembe.2009.06.002
Whiteley N. M., Taylor E. W. (1992). Oxygen and Acid-Base Disturbances in the Hemolymph of the Lobster Homarus gammarus During Commercial Transport and Storage. J. Crustac. Biol. 12, 19–30. doi:10.2307/1548715
Whiteley N. M., Egginton S., Taylor E. W., Raven J. A. (1999). Acid-base Regulations in Crustaceans: The Role of Bicarbonate Ions. Cambridge: Cambridge University Press.
Whiteley N. M. (2011). Physiological and Ecological Responses of Crustaceans to Ocean Acidification. Mar. Ecol. Prog. Ser. 430, 257–271. doi:10.3354/meps09185
Whiteley N. M, Suckling C. C., Ciotti B. J., Brown J., McCarthy I. D., Gimenez L., et al. (2018). Sensitivity to Near-Future CO2 Conditions in Marine Crabs Depends on Their Compensatory Capacities for Salinity Change. Sci. Rep. 8, 15639. doi:10.1038/s41598-018-34089-0
Wickham H. (2016). ggplot2: Elegant Graphics for Data Analysis. New York: Springer-Verlag. Available at: https://ggplot2.tidyverse.org.
Wiltshire K. H., Malzahn A. M., Wirtz K., Greve W., Janisch S., Mangelsdorf P., et al. (2008). Resilience of North Sea Phytoplankton Spring Bloom Dynamics: An Analysis of Long-Term Data at Helgoland Roads. Limnol. Oceanogr. 53, 1294–1302. doi:10.4319/lo.2008.53.4.1294
Keywords: climate change, ocean warming, thermal tolerance, early life stages, decapod
Citation: Leiva L, Tremblay N, Torres G, Boersma M, Krone R and Giménez L (2022) European Lobster Larval Development and Fitness Under a Temperature Gradient and Ocean Acidification. Front. Physiol. 13:809929. doi: 10.3389/fphys.2022.809929
Received: 05 November 2021; Accepted: 20 June 2022;
Published: 14 July 2022.
Edited by:
Zbigniew Adamski, Adam Mickiewicz University in Poznań, PolandReviewed by:
Ilie S. Racotta, Centro de Investigación Biológica del Noroeste (CIBNOR), MexicoBasile Michaelidis, Aristotle University of Thessaloniki, Greece
Copyright © 2022 Leiva, Tremblay, Torres, Boersma, Krone and Giménez. This is an open-access article distributed under the terms of the Creative Commons Attribution License (CC BY). The use, distribution or reproduction in other forums is permitted, provided the original author(s) and the copyright owner(s) are credited and that the original publication in this journal is cited, in accordance with accepted academic practice. No use, distribution or reproduction is permitted which does not comply with these terms.
*Correspondence: Laura Leiva, laura.leiva@awi.de