- Geomicrobiology, GEOMAR Helmholtz Centre for Ocean Research Kiel, Kiel, Germany
Researchers have recognized the potential of enzymes and metabolic pathways hidden among the unseen majority of Earth’s microorganisms for decades now. Most of the microbes expected to colonize the seafloor and its subsurface are currently uncultured. Thus, their ability and contribution to element cycling remain enigmatic. Given that the seafloor covers ∼70% of our planet, this amounts to an uncalled potential of unrecognized metabolic properties and interconnections catalyzed by this microbial dark matter. Consequently, a tremendous black box awaits discovery of novel enzymes, catalytic abilities, and metabolic properties in one of the largest habitats on Earth. This mini review summarizes the current knowledge of cultivation-dependent and -independent techniques applied to seafloor habitats to unravel the role of the microbial dark matter. It highlights the great potential that combining microbiological and biogeochemical data from in situ experiments with molecular tools has for providing a holistic understanding of bio-geo-coupling in seafloor habitats and uses hydrothermal vent systems as a case example.
Introduction
The ocean’s seafloor covers ∼70% of our planet’s surface and is vastly underexplored. Through its pivotal role for processing deposited material in marine sediments, the seafloor is critically involved in the extent to which carbon sequestration, nutrient recycling, carbonate dissolution and methane production occur (cf. Middelburg, 2018; LaRowe et al., 2020). Most of the seafloor is in the deep-sea and is hallmarked by hostile conditions, i.e., no light, high pressure, food scarcity, and is mostly characterized by comparatively low turnover rates (Middelburg et al., 1993). Although hydrothermal deep-sea vent ecosystems can be associated with even more extreme conditions, such as high temperatures or the presence of toxic compounds (Perner et al., 2014; McDermott et al., 2018), the emitted inorganic energy sources and chemosynthetic microbes capable of coping with local extreme conditions transform deep-sea hydrothermal vents into hot spots of activity. Venting is also a significant metal source to the ocean, with metal-organic complexation facilitating long-distance transport and potentially impacting primary production in the ocean’s surface (Sander and Koschinsky, 2011; Resing et al., 2015; Fitzsimmons et al., 2017; Ardyna et al., 2019). Additionally, hydrothermal environments are relevant for providing bioactive trace metals (Li et al., 2014; Cohen et al., 2021) and organic carbon (Toner et al., 2009; Bennett et al., 2015; Longnecker et al., 2018), and give insights into the origin of life and its limits (Martin et al., 2008).
Microbes make up most of the total biomass on Earth. However, the majority of prokaryotic cells resist cultivation and remain uncharacterized (Lloyd et al., 2018; Zamkovaya et al., 2021). It is estimated that this uncultured prokaryotic majority, often referred to as microbial dark matter, accounts for up to 91 and 96% of uncultured bacteria and 87 and 96% of uncultured archaea in marine sediments and hydrothermal vents, respectively (Lloyd et al., 2018). Sequencing of prokaryotic (meta)genomes has demonstrated that up to 40% of annotated genes cannot be allocated to a known or predicted function (Baric et al., 2016) and only as little as 16% of ocean metagenomic DNA encoding hypothetical proteins could be linked to proteins with an experimentally verified function (Sunagawa et al., 2015). One way to address this sequence-based limitation is the development of novel computational approaches like, e.g., the CSBFinder-S software. It allows identification of operon structures by inferring conserved synthetic blocks (CSBs), providing a functional context for unassignable enzymes (Svetlitsky et al., 2020).
So far, meta’omics has given us valuable insights into the taxonomic diversity, metabolic potential and gene expression patterns of microbial communities from extreme seafloor habitats (Fortunato and Huber, 2016 and references therein). Albeit, activity-based screening of metagenomic libraries is the only methodology that currently allows detection of entirely novel enzymes from known and unknown microbes for which homologies to known motifs lack and is a promising approach to overcome shortcomings associated with sequence-based strategies (Handelsman, 2004; Böhnke and Perner, 2014; Adam and Perner, 2018; Pushkarev et al., 2018). However, recombinant expression of metagenomic fragments in a surrogate host can be troublesome due to manifold reasons (divergent codon usage, translation, correct folding etc.), often leading to low hit rates that require high screening throughput which is the reason why functional metagenomic approaches might be very time-consuming and cost intensive (Perner et al., 2011b). Another way to study yet uncultured microbes is to perform the corresponding investigations directly in the natural habitat, i.e., in situ. Here, the main challenge is not only to further develop sensor technology and to optimize the collection and preservation of sample material, but also to provide technologies that synchronize in situ microbiological and geochemical investigations in space and time (Figure 1).
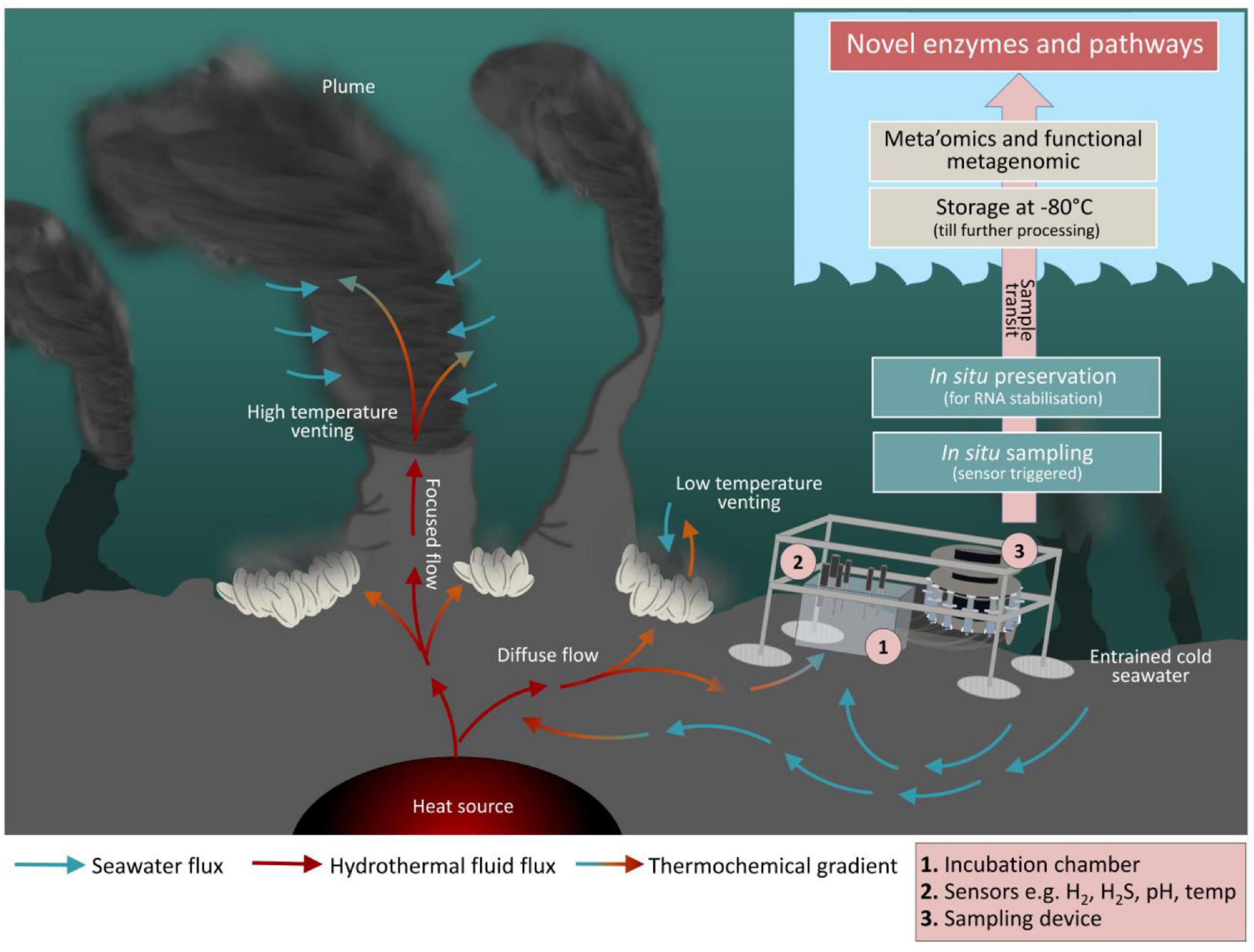
Figure 1. Future perspective for hydrothermal vent in situ incubations. A mini chamber lander and the related work flow is shown next to a hydrothermal vent. The mini chamber is equipped with various sensors to measure the local environmental parameters like O2, H2, H2S, pH, temperature, redox potential, and conductivity. Sampling may take place as a time series and/or controlled through the change in certain environmental parameters. Moreover, it is possible to simulate particular what-if scenarios as the syringe samplers may also function as injectors. This allows for manipulation of certain environmental conditions in the chamber as incubation proceeds. The subsamples collected during incubation are filtered and preserved in situ using appropriated fixation reagents. Finally, once on board, filters are stored at –80°C until further processing in the home laboratory. Mini chamber lander systems comparable to the here illustrated one have successfully been used to investigate benthic fluxes at the sediment-water interface zone of shallow waters or in the deep-sea (Thoms et al., 2018; Vonnahme et al., 2020; Kononets et al., 2021 and references therein). However, we are not aware of any published work that has reported data generated from the here presented approach where in situ incubation at hydrothermal vent environments with sensor-triggered sampling, in situ preservation, and subsequent microbiological analyses has been combined to elucidate interrelationships and interdependencies between abiotic factors and the biological world.
The Not Yet Cultivated Microbial Majority and Its Potential for Element Cycling at Hydrothermal Vent Habitats
Deep-sea hydrothermal vent environments form along spreading ridges, where hot, highly reduced hydrothermal fluids mix with cold, oxygenated seawater, thereby creating steep thermal and chemical gradients. Chemosynthetic microorganisms exploit this thermodynamic disequilibrium by generating energy through redox reactions potentially fueling autotrophic carbon fixation. Since the discovery of hydrothermal vents (Ballard, 1977; Corliss et al., 1979), great cultivation efforts have been made to describe metabolic activities and physiological properties of respective microbes (reviewed in Dick, 2019). Cultivation is irreplaceable and includes (i) traditional and steadily improved techniques on liquid or solid media (cf. Reysenbach and Götz, 2001; Hansen and Perner, 2015; Zhang et al., 2018; Zeng et al., 2021 and references therein), (ii) gradient tube incubations (Emerson and Moyer, 1997), enrichments (iii) in bio-electrochemical systems (Pillot et al., 2018), (iv) on in situ enrichment carriers (Stokke et al., 2020), or (v) possibly–in the near future–even on synthetically grown hydrothermal vents (Barge and White, 2017; Martinez et al., 2019; Sanchez, 2021), and high-pressure laboratory techniques (Kato, 2011). Information from meta’omic data holds great promise to further improve the cultivation success by guiding the development of new cultivation technologies and strategies that are more responsive to the requirements of uncultured lineages (Gutleben et al., 2018). Once strains are in culture, the next step is the generation of a pure culture, but isolation of microbes is far from trivial. Strains often tend to grow in close co-culture with other strains and a variety of different isolation strategies include plating techniques (cf. Sass and Perner, 2020), role-tube isolations (cf. Zeng et al., 2013), dilution to extension approaches (cf. Adam et al., 2021), single cell separation micro tweezer technologies (Fröhlich and König, 2000; cf. Sass et al., 2020), flow cytometry (Ferrari et al., 2012), diffuse chamber incubation (Kaeberlein et al., 2002) etc. These approaches have resulted in the description of some hundred microbial species with hydrothermal origin (Jebbar et al., 2015). Nevertheless, sequence-based metagenome studies disclose a large discrepancy between microbes present in a certain environment and those that are cultivable (Rinke et al., 2013; Hug et al., 2016; Zamkovaya et al., 2021). With respect to hydrothermal systems and marine sediments, this corresponds to 4 and 9% cultured bacteria and 4 and 13% cultured archaea, respectively (Lloyd et al., 2018). Despite technical progress and relentless efforts, hydrothermal vents are still among the ecosystems with particularly high numbers of uncultivated representatives (Lloyd et al., 2018). Meta’omic studies of hydrothermal vent habitats suggest that the functional differences between closely related microbial species or strains are substantial (Hug et al., 2016; Dombrowski et al., 2017). This highlights an unprecedented potential for various new metabolic pathways and enzyme functions hidden among the non-cultured majority of hydrothermal vent microbes (Zamkovaya et al., 2021). In order to cope with the ever-increasing amount of sequence information and to prevent the gap between physiological and sequence-based information from widening, (high-throughput) approaches linking sequences with functions urgently need to be further developed and advanced.
Metagenomics: Towards Understanding the Metabolic Microbial Network
Metagenomics refers to the entire genetic information of a given ecosystem (Handelsman et al., 1998). The original metagenomic approach was based on sequence- or function-based screening of metagenomic libraries that contained cloned environmental DNA (Lam et al., 2015). In 2004, the large marine whole genome shotgun sequencing project of the Sargasso Sea, provided, for the first time, a glimpse into the complex microbial community compositions of ocean habitats (Venter et al., 2004), pioneering future metagenome projects. The progress in next generation sequencing technologies has been rapid and together with bioinformatic tool development has allowed the subfields of metatranscriptomics and metaproteomics to further revolutionize meta’omic research (Simon and Daniel, 2011), as gene expression and protein profiles now enable insights into active metabolic processes and functional adaptations (Wilmes et al., 2015; Shakya et al., 2019). In this context, the term functional metagenomics has popped up frequently. This is rather misleading, as this term was originally used for function-based screening of metagenomic libraries seeking specific enzyme activities or valuable compounds (Handelsman, 2004). In the following we use the term functional metagenomics as it was initially coined.
However, high-throughput meta’omic approaches nowadays result in the rapid accumulation of DNA, RNA, and protein sequences, but current databases only allow the assignment of candidate functions based on homologs of already known motifs (Daniel, 2005). Thus, the vast majority of predicted enzyme functions have never been experimentally proven. Indeed, about one-third of the genes found in genomes of cultured and uncultured prokaryotes cannot even be assigned a predicted function due to the lack of homologies (Lloyd et al., 2018). One possible approach suited to verify if a predicted function is true is to clone and express targeted genes in a surrogate host (Yang et al., 2016; Danso et al., 2018; Oppermann et al., 2019). However, one major drawback of this strategy is that the original gene proximity and thus relevant chaperones, transcriptional regulators and/or activators are missing, likely causing corresponding gene products to remain inactive (Böhnke and Perner, 2017). Although the use of large insert metagenomic libraries has the potential to counteract some of these challenges, problems with heterologous gene expression in the surrogate host, e.g., failed gene expression and incorrect post-transcriptional processing, remain one of the major limitations of functional metagenomic approaches (Perner et al., 2011b; Johnson et al., 2017). The use of custom expression strains, alternative vector systems, ionic liquids, or even in vitro recombinant transcription systems are promising techniques to mitigate these shortcomings (Lam et al., 2015; Kinfu et al., 2017; Mital et al., 2021).
Implementing a functional metagenomic approach requires two further major challenges to be overcome. First, there is the need to construct metagenomic libraries whereby isolation of high-quality environmental DNA is critical for successful cloning. The second major bottleneck is the often very time consuming and tedious establishments of high-throughput screening methods. A large range of biotechnologically motivated screening technologies for identifying novel biocatalysts or valuable biomolecules with industrial, commercial, clinical or bioremediational applications from uncultured microbes has identified proteases, oxidoreductases, esterases, amylases, phosphatases, chitinases, cellulases, glycosyltransferases, and decarboxylases (cf. Perner et al., 2011b; Rabausch et al., 2013; Berini et al., 2017; Johnson et al., 2017). However, functional metagenomic approaches with ecologically oriented objectives are extremely rare; although some enzymes discovered out of a biotechnological interest may also offer insights into ecologically relevant metabolic processes. Recently, one of the few purely ecologically and biogeochemically motivated functional screening approaches available targeted the distribution of active ribulose-1,5-bisphosphate carboxylases (RubisCO) at different hydrothermal vents (Böhnke and Perner, 2019). The study managed to place the identified RubisCOs (and respective uncultivated microbes) into an ecological context and demonstrated some possible RubisCO-protein interactions with neighboring gene products (Böhnke and Perner, 2017). As part of this work, some of the previously annotated “hypothetical proteins with unknown functions,” could be assigned the probable role as RubisCO transcriptional regulators and post-translational activators or repressors.
Additionally, a second ecologically motivated function-based screen was developed that also targets RubisCO activity (Varaljay et al., 2016). Since Varaljay et al. (2016) used a different host-vector system, this heterologous complementation based functional metagenomic screen likely expands the spectrum of detectable active RubisCOs (Varaljay et al., 2016). Another ecologically and biogeochemically motivated functional metagenomic approach focused on hydrogenase activities (Adam and Perner, 2017). The screening detected three H2-uptake expressing active metagenomic clones without any known hydrogenase-encoding genes or motifs on their DNA insert (Adam and Perner, 2018) suggesting novel hydrogenases. The discovery of heliorhodopsin, a globally abundant and widely distributed light-sensing rhodopsin, has also been enabled by functional metagenomics (Pushkarev et al., 2018). These studies highlight the tremendous diversity of currently unknown dark matter proteins and underline the urgent need for developing more novel screening methods for targeting specific enzymatic activities of unknown organisms. This methodology allows a window into the metabolic network of the uncultured microbes and their catalytic ability in biogeochemical cycling of key elements.
Current Challenges and Future Perspectives for in situ Technologies at the Seafloor
Marine microbial communities hold a central role as drivers of major biogeochemical processes, impacting ecosystem functioning far beyond the oceans (Falkowski et al., 2008). Research into these microbial consortia and the processes they mediate is, however, often constrained by technical capabilities, as is particularly evident in deep-sea research (Fortunato et al., 2021). Thus, sampling hard accessible deep-sea environments is already a technical and logistical challenge, requiring the development of specialized underwater devices (Liang et al., 2021; Paulus, 2021). Over the past decades a variety of ocean deployable sampling instrumentation have been developed (McQuillan and Robidart, 2017). Yet, only a few of them are suited to retrieve samples from extreme deep-sea habitats and are able to withstand the high pressures and corrosive hot fluids (Reysenbach and Götz, 2001; Liang et al., 2021). Transporting the samples from the deep-sea through the water column to the research vessel laboratory poses further inherent limitations as the samples are exposed to physico-chemical changes (e.g., changes in pressure, temperature, light, pH, redox state etc.) altering the compositions and thus biasing subsequent analysis (Edgcomb et al., 2016). Chemical composition of sampled hydrothermal fluids can change dramatically if in situ pressure is not maintained, resulting in degassing and the loss of volatile species and distorting microbial activities and metabolic rates (McNichol et al., 2016). Gas-tight sampling devices are used to mitigate this effect and prevent outgassing (Seewald et al., 2002; Butterfield et al., 2004; Miyazaki et al., 2017; Wu et al., 2018; Garel et al., 2019; Wang et al., 2020). A long and often variable lag time during ascent may change redox reactions, introducing artifacts in subsequent analyses despite the usage of pressure maintaining sampling devices (Fortunato et al., 2021). Once on board, the samples are subjected to atmospheric pressure for ex situ filtration, likely causing cell lyses and release of RNA and DNA molecules (Edgcomb et al., 2016). Extracellular DNA and RNA from lysed cells can only partially be bound and recovered by filtration (Liang and Keeley, 2013), thereby losing parts of the unknown microbial community. Unpreserved biological material is also very labile and starts to degrade within minutes (RNA and proteins) or hours to days (cells and DNA), further biasing samples (Ottesen, 2016). Indeed, a comparative study of in situ and shipboard RNA stable isotope probing (RNA-SIP) experiments showed that microbial communities are significantly affected by the effects of depressurization and sample processing delays, resulting in a shift of the community structure and metabolic function (Fortunato et al., 2021). In situ preservation is one approach that has successfully been used to overcome limitations associated with sample transit (Edgcomb et al., 2016; Fortunato et al., 2021). But devices designed for filtration and integrated subsequent preservation are still rare (reviewed in Ottesen, 2016). They include the Suspended Particulate Rosette V2 (SUPR-V2) System (Breier et al., 2014), the Biological Osmo Sampling System (BOSS) (Robidart et al., 2013), and the Fixation Filter Unit (FF3) (Taylor et al., 2015).
The more information on habitat specific physicochemical characteristics available, the more value can be deduced from generated meta’omic datasets. This is essential if aiming to understand the role of microbes for ocean ecosystem functioning. Deep-sea sensors are efficient tools for observing local geochemistry, allowing real-time monitoring of certain key chemical variables such as pH, dissolved H2, H2S, CH4, CO2, and dissolved inorganic nutrients (Luther et al., 2001; Moore et al., 2009; Petersen et al., 2011; Wankel et al., 2011; Perner et al., 2013; Daniel et al., 2020; Gros et al., 2021; Liang et al., 2021; Mowlem et al., 2021). However, technical limitations require that various chemical parameters still have to be determined ex situ (Mowlem et al., 2021). Although in situ filtration allows reduction of chemical alteration caused by precipitation and/or adsorption of some dissolved elements during transit from the seafloor to the ship’s research laboratory, it is evident that the most representative data on deep-sea fluid chemistry would be provided by direct in situ measurements (Sievert and Vetriani, 2012; Cotte et al., 2015). Thus, future efforts must be directed toward further advancing existing sensors (more precision, robustness, serialization and standardization) and establishing novel sensor technologies.
Technological advances in the past decade have enabled the development of a limited set of samplers capable of performing in situ experiments directly in the deep-sea, pioneering future biogeochemical studies in deep-sea habitats. Respective devices have successfully been used to perform in situ tracer incubations (Edgcomb et al., 2016), RNA-SIP experiments (Fortunato et al., 2021), molecular analytical techniques (Scholin et al., 2017), and extraction of organic compounds (Grandy et al., 2020). This has impressively demonstrated that in situ experiments can provide a window into the seafloor microbial consortia, metabolic mechanisms and transformations. To obtain a more complete understanding of microbial community dynamics, functions and influences on ocean processes, microbiology and geochemistry must be sampled simultaneously. Automated mini chamber lander systems have a great potential as they allow time series sampling in response to changes of environmental conditions, e.g., O2, H2S etc. (Figure 1). Furthermore, the possibility of injecting selected chemical compounds into the in situ incubation chamber could be used to simulate different what-if-scenarios. Thereby they can contribute to forecasting potential climate change impacts on the deep-sea microbes and the biogeochemical processes they mediate. Embedded in a holistic approach (Figure 2), in situ microbiological and biogeochemical analyses conducted in spatial and temporal proximity to each other can provide a more comprehensive picture of what features influence overall biogeochemical fluxes. This in turn improves the basis for building predictive models of how deep-sea microbial consortia contribute to global biogeochemical cycles.
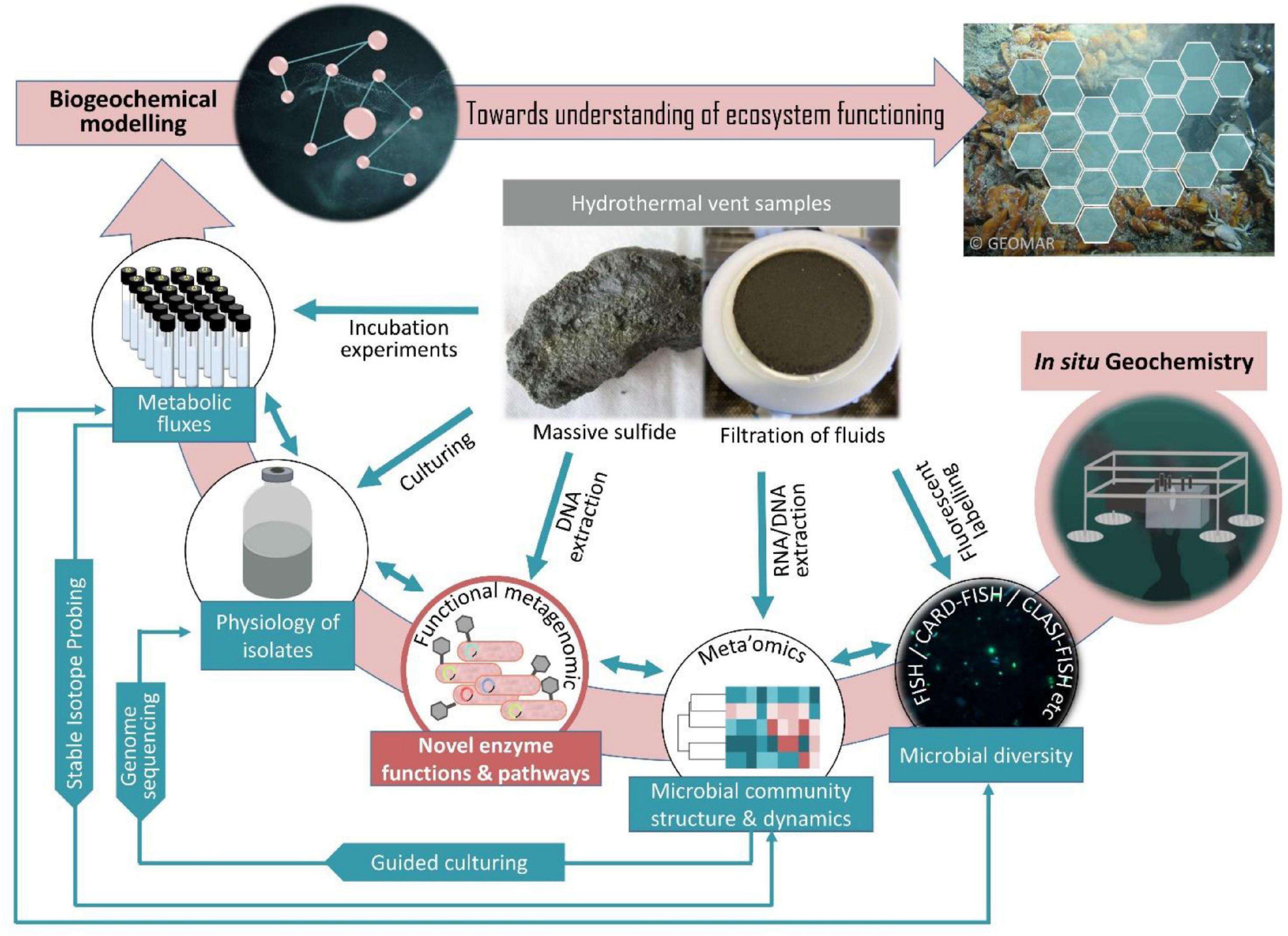
Figure 2. A holistic approach to study the structure and function of microbial consortia in hydrothermal vents. The overview shows the approaches that should be considered and combined, if aiming at an omni-directional insight into hydrothermal vent ecosystem functioning, without neglecting the yet uncultivable majority of microorganisms.
Biogeochemical modeling is successfully used to determine (i) element flux rates of trace metals like, e.g., Fe, Mn, Ni, Cu, Co, Cd and Zn (reviewed in Homoky et al., 2016; cf. Somes et al., 2021), (ii) particulate organic material (POM) reactivity (reviewed in Lessin et al., 2018), (iii) thermodynamics (cf. Perner et al., 2011a) and (iv) energetics (cf. Böhnke et al., 2019), there by enhancing our theoretical and quantitative understanding of microbial and geochemical interactions (Dick, 2019). Only a few biogeochemical models have been established in recent years that allow the linkage between microbial biogeochemical rate measurements and meta’omic data, making key unknown physiological parameters, such as kinetic properties, transcription and translation rates, and mRNA and protein degradation rates recognizable (Reed et al., 2014; Louca et al., 2016). Such models have great potential and hold promise to unprecedented predictions about the role of ubiquitous microorganisms in mediating global element cycling.
Conclusion
The current understanding of the contribution of seafloor microbes to global biogeochemical cycles, metabolic fluxes and ecosystem functions is primarily aligned with what we known from culturable microbes. The cultured microbes, however, only represent a minor fraction of the total microbial vent community. This shows that our current understanding is vastly incomplete. Cultivation-independent approaches including in situ technologies, biogeochemical rate measurements, functional metagenomics, meta’omics, and biogeochemical modeling are promising tools that have already been used to effectively complement cultivation-dependent methods. Clearly, no single technology will provide full access to the vast potential of novel metabolic pathways hidden among the majority of uncultured microorganisms. The great challenge, but also the most promising approach for the future, can only lie in harnessing the strengths of available cultivation-dependent and cultivation-independent tools and smartly combining them in a holistic multidisciplinary approach. Here, continuing the development of existing in situ technologies and experimentation, but also the establishment of completely new ones, is of major importance and will significantly drive progress toward opening the window into previously inaccessible microbial physiologies of the microbial dark matter.
Author Contributions
SB and MP wrote the manuscript. Both authors contributed to the article and approved the submitted version.
Conflict of Interest
The authors declare that the research was conducted in the absence of any commercial or financial relationships that could be construed as a potential conflict of interest.
Publisher’s Note
All claims expressed in this article are solely those of the authors and do not necessarily represent those of their affiliated organizations, or those of the publisher, the editors and the reviewers. Any product that may be evaluated in this article, or claim that may be made by its manufacturer, is not guaranteed or endorsed by the publisher.
Acknowledgments
We thank all colleagues and collaborators for their fruitful discussion on functional metagenomics and in situ experimentations. Furthermore, we greatly appreciate the help of the captains and crews of the research vessels and underwater vehicles for helping us to obtain our samples over the years.
References
Adam, N., Han, Y., Laufer-Meiser, K., Bährle, R., Schwarz-Schampera, U., Schippers, A., et al. (2021). Deltaproteobacterium strain KaireiS1, a mesophilic, hydrogen-oxidizing and sulfate-reducing bacterium from an inactive deep-sea hydrothermal chimney. Front. Microbiol. 12:686276. doi: 10.3389/fmicb.2021.686276
Adam, N., and Perner, M. (2017). Activity-based screening of metagenomic libraries for hydrogenase enzymes. Methods Mol. Biol. 1539, 261–270.
Adam, N., and Perner, M. (2018). Novel hydrogenases from deep-sea hydrothermal vent metagenomes identified by a recently developed activity-based screen. ISME J. 12, 1225–1236. doi: 10.1038/s41396-017-0040-6
Ardyna, M., Lacour, L., Sergi, S., d’Ovidio, F., Sallee, J. B., Rembauville, M., et al. (2019). Hydrothermal vents trigger massive phytoplankton blooms in the Southern Ocean. Nat. Commun. 10:2451. doi: 10.1038/s41467-019-09973-6
Barge, L. M., and White, L. M. (2017). Experimentally testing hydrothermal vent origin of life on enceladus and other icy/ocean worlds. Astrobiology 17, 820–833. doi: 10.1089/ast.2016.1633
Baric, R. S., Crosson, S., Damania, B., Miller, S. I., and Rubin, E. J. (2016). Next-generation high-throughput functional annotation of microbial genomes. mBio 7, e1245–e1216. doi: 10.1128/mBio.01245-16
Bennett, S. A., Van Dover, C., Breier, J. A., and Coleman, M. (2015). Effect of depth and vent fluid composition on the carbon sources at two neighboring deep-sea hydrothermal vent fields (Mid-Cayman Rise). Deep-Sea Res. Part I-Oceanogr. Res. Papers 104, 122–133. doi: 10.1016/j.dsr.2015.06.005
Berini, F., Casciello, C., Marcone, G. L., and Marinelli, F. (2017). Metagenomics: novel enzymes from non-culturable microbes. FEMS Microbiol. Lett. 364:fnx211. doi: 10.1093/femsle/fnx211
Böhnke, S., and Perner, M. (2014). A function-based screen for seeking RubisCO active clones from metagenomes: novel enzymes influencing RubisCO activity. ISME J. 9, 735–745. doi: 10.1038/ismej.2014.163
Böhnke, S., and Perner, M. (2017). Unraveling RubisCO form I and form II regulation in an uncultured organism from a deep-sea hydrothermal vent via metagenomic and mutagenesis studies. Front. Microbiol. 8:1303. doi: 10.3389/fmicb.2017.01303
Böhnke, S., and Perner, M. (2019). Seeking active RubisCOs from the currently uncultured microbial majority colonizing deep-sea hydrothermal vent environments. ISME J. 13, 2475–2488. doi: 10.1038/s41396-019-0439-3
Böhnke, S., Sass, K., Gonnella, G., Diehl, A., Kleint, C., Bach, W., et al. (2019). Parameters governing the community structure and element turnover in Kermadec volcanic ash and hydrothermal fluids as monitored by inorganic electron donor consumption, autotrophic CO2 fixation and 16S tags of the transcriptome in incubation experiments. Front. Microbiol. 10:2296. doi: 10.3389/fmicb.2019.02296
Breier, J. A., Sheik, C. S., Gomez-Ibanez, D., Sayre-McCord, R. T., Sanger, R., Rauch, C., et al. (2014). A large volume particulate and water multi-sampler with in situ preservation for microbial and biogeochemical studies. Deep-Sea Res. Part I-Oceanogr. Res. Papers 94, 195–206. doi: 10.1016/j.dsr.2014.08.008
Butterfield, D. A., Roe, K. K., Lilley, M. D., Huber, J. A., Baross, J. A., Embley, R. W., et al. (2004). “Mixing, reaction and microbial activity in the sub-seafloor revealed by temporal and spatial variation in diffuse flow vents at axial volcano,” in The Subseafloor Biosphere at Mid-Ocean Ridges, eds W. S. D. Wilcock, E. F. Delong, D. S. Kelley, J. A. Barass, and S. C. Cary (Washington, DC: AGU), 269–289. doi: 10.1029/144gm17
Cohen, N. R., Noble, A. E., Moran, D. M., McIlvin, M. R., Goepfert, T. J., Hawco, N. J., et al. (2021). Hydrothermal trace metal release and microbial metabolism in the northeastern Lau Basin of the South Pacific Ocean. Biogeosciences 18, 5397–5422. doi: 10.5194/bg-18-5397-2021
Corliss, J. B., Dymond, J., Gordon, L. I., Edmond, J. M., von Herzen, R. P., Ballard, R. D., et al. (1979). Submarine thermal springs on the Galápagos Rift. Science 203, 1073–1083. doi: 10.1126/science.203.4385.1073
Cotte, L., Waeles, M., Pernet-Coudrier, B., Sarradin, P. M., Cathalot, C., and Riso, R. D. (2015). A comparison of in situ vs. ex situ filtration methods on the assessment of dissolved and particulate metals at hydrothermal vents. Deep-Sea Res. Part I 105, 186–194. doi: 10.1016/j.dsr.2015.09.005
Daniel, A., Laës-Huon, A., Barus, C., Beaton, A. D., Blandfort, D., Guigues, N., et al. (2020). Toward a harmonization for using in situ nutrient sensors in the marine environment. Front. Marin. Sci. 6:773. doi: 10.3389/fmars.2019.00773
Danso, D., Schmeisser, C., Chow, J., Zimmermann, W., Wei, R., Leggewie, C., et al. (2018). New insights into the function and global distribution of polyethylene terephthalate (PET)-degrading Bacteria and enzymes in marine and terrestrial metagenomes. Appl. Environ. Microbiol. 84, e02773–17 doi: 10.1128/AEM.02773-17
Dick, G. J. (2019). The microbiomes of deep-sea hydrothermal vents: distributed globally, shaped locally. Nat. Rev. Microbiol. 17, 271–283. doi: 10.1038/s41579-019-0160-2
Dombrowski, N., Seitz, K. W., Teske, A. P., and Baker, B. J. (2017). Genomic insights into potential interdependencies in microbial hydrocarbon and nutrient cycling in hydrothermal sediments. Microbiome 5:106. doi: 10.1186/s40168-017-0322-2
Edgcomb, V. P., Taylor, C., Pachiadaki, M. G., Honjo, S., Engstrom, I., and Yakimov, M. (2016). Comparison of Niskin vs. in situ approaches for analysis of gene expression in deep Mediterranean Sea water samples. Deep-Sea Res. Part II 129, 213–222. doi: 10.1016/j.dsr2.2014.10.020
Emerson, D., and Moyer, C. (1997). Isolation and characterization of novel iron-oxidizing bacteria that grow at circumneutral pH. Appl. Environ. Microbiol. 63, 4784–4792. doi: 10.1128/aem.63.12.4784-4792.1997
Falkowski, P. G., Fenchel, T., and Delong, E. F. (2008). The microbial engines that drive Earth’s biogeochemical cycles. Science 320, 1034–1039. doi: 10.1126/science.1153213
Ferrari, B. C., Winsley, T. J., Bergquist, P. L., and van Dorst, J. (2012). “Flow cytometry in environmental microbiology: A rapid approach for the isolation of single cells for advanced molecular biology analysis,” in Microbial Systems Biology: Methods and Protocols, ed. A. Navid (New Jersey, NJ: Humana Press Inc), 3–26. doi: 10.1007/978-1-61779-827-6_1
Fitzsimmons, J. N., John, S. G., Marsay, C. M., Hoffman, C. L., Nicholas, S. L., Toner, B. M., et al. (2017). Iron persistence in a distal hydrothermal plume supported by dissolved-particulate exchange. Nat. Geosci. 10, 195–201. doi: 10.1038/ngeo2900
Fortunato, C. S., Butterfield, D. A., Larson, B., Lawrence-Slavas, N., Algar, C. K., Zeigler Allen, L., et al. (2021). Seafloor incubation experiment with deep-sea hydrothermal vent fluid reveals effect of pressure and lag time on autotrophic microbial communities. Appl. Environ. Microbiol. 87, e78–e21. doi: 10.1128/AEM.00078-21
Fortunato, C. S., and Huber, J. A. (2016). Coupled RNA-SIP and metatranscriptomics of active chemolithoautotrophic communities at a deep-sea hydrothermal vent. ISME J. 10, 1925–1938. doi: 10.1038/ismej.2015.258
Fröhlich, J., and König, H. (2000). New techniques for isolation of single prokaryotic cells. FEMS Microbiol. Rev. 24, 567–572. doi: 10.1111/j.1574-6976.2000.tb00558.x
Garel, M., Bonin, P., Martini, S., Guasco, S., Roumagnac, M., Bhairy, N., et al. (2019). Pressure-retaining sampler and high-pressure systems to study deep-sea microbes under in situ conditions. Front. Microbiol. 10:453. doi: 10.3389/fmicb.2019.00453
Grandy, J. J., Onat, B., Tunnicliffe, V., Butterfield, D. A., and Pawliszyn, J. (2020). Unique solid phase microextraction sampler reveals distinctive biogeochemical profiles among various deep-sea hydrothermal vents. Sci. Rep. 10:1360. doi: 10.1038/s41598-020-58418-4
Gros, J., Schmidt, M., Linke, P., Dötsch, S., Triest, J., Martínez-Cabanas, M., et al. (2021). Quantification of dissolved CO2 plumes at the Goldeneye CO2-release experiment. Int. J. Greenhouse Gas Control 109:103387. doi: 10.1016/j.ijggc.2021.103387
Gutleben, J., Chaib, De Mares, M., van Elsas, J. D., Smidt, H., Overmann, J., et al. (2018). The multi-omics promise in context: from sequence to microbial isolate. Crit. Rev. Microbiol. 44, 212–229. doi: 10.1080/1040841X.2017.1332003
Handelsman, J. (2004). Metagenomics: application of genomics to uncultured microorganisms. Microbiol. Mol. Biol. Rev. 68, 669–685. doi: 10.1128/MMBR.68.4.669-685.2004
Handelsman, J., Rondon, M. R., Brady, S. F., Clardy, J., and Goodman, R. M. (1998). Molecular biological access to the chemistry of unknown soil microbes: a new frontier for natural products. Chem. Biol. 5, R245–R249. doi: 10.1016/s1074-5521(98)90108-9
Hansen, M., and Perner, M. (2015). A novel hydrogen oxidizer amidst the sulfur-oxidizing Thiomicrospira lineage. ISME J. 9, 696–707. doi: 10.1038/ismej.2014.173
Homoky, W. B., Weber, T., Berelson, W. M., Conway, T. M., Henderson, G. M., van Hulten, M., et al. (2016). Quantifying trace element and isotope fluxes at the ocean-sediment boundary: a review. Philos. Trans. Royal Soc. A 374:20160246. doi: 10.1098/rsta.2016.0246
Hug, L. A., Baker, B. J., Anantharaman, K., Brown, C. T., Probst, A. J., Castelle, C. J., et al. (2016). A new view of the tree of life. Nat. Microbiol. 1:16048.
Jebbar, M., Franzetti, B., Girard, E., and Oger, P. (2015). Microbial diversity and adaptation to high hydrostatic pressure in deep-sea hydrothermal vents prokaryotes. Extremophiles 19, 721–740. doi: 10.1007/s00792-015-0760-3
Johnson, J., Jain, K., and Madamwar, D. (2017). “Functional metagenomics: exploring nature’s gold mine,” in Current Developments in Biotechnology and Bioengineering, eds P. Gunasekaran, S. Noronha, and A. Pandey (Amsterdam: Elsevier), 27–43.
Kaeberlein, T., Lewis, K., and Epstein, S. S. (2002). Isolating “uncultivable” microorganisms in pure culture in a simulated natural environment. Science 296, 1127–1129. doi: 10.1126/science.1070633
Kato, C. (2011). “Cultivation methods for piezophiles,” in Extremophiles Handbook, ed. K. Horikoshi (Japan: Springer), 719–726. doi: 10.1007/978-4-431-53898-1_34
Kinfu, B. M., Jahnke, M., Janus, M., Besirlioglu, V., Roggenbuck, M., Meurer, R., et al. (2017). Recombinant RNA polymerase from Geobacillus sp. GHH01 as tool for rapid generation of metagenomic RNAs using in vitro technologies. Biotechnol. Bioeng. 114, 2739–2752. doi: 10.1002/bit.26436
Kononets, M., Tengberg, A., Nilsson, M., Ekeroth, N., Hylén, A., Robertson, E. K., et al. (2021). In situ incubations with the Gothenburg benthic chamber landers: applications and quality control. J. Marin. Syst. 214:103475. doi: 10.1016/j.jmarsys.2020.103475
Lam, K. N., Cheng, J., Engel, K., Neufeld, J. D., and Charles, T. C. (2015). Current and future resources for functional metagenomics. Front. Microbiol. 6:1196. doi: 10.3389/fmicb.2015.01196
LaRowe, D. E., Arndt, S., Bradley, J. A., Burwicz, E., Dale, A. W., and Amend, J. P. (2020). Organic carbon and microbial activity in marine sediments on a global scale throughout the Quaternary. Geochimica Et Cosmochimica Acta 286, 227–247. doi: 10.1016/j.gca.2020.07.017
Lessin, G., Artioli, Y., Almroth-Rosell, E., Blackford, J. C., Dale, A. W., Glud, R. N., et al. (2018). Modelling marine sediment biogeochemistry: current knowledge gaps, challenges, and some methodological advice for advancement. Front. Marin. Sci. 5:19. doi: 10.3389/fmars.2018.00019
Li, M., Toner, B. M., Baker, B. J., Breier, J. A., Sheik, C. S., and Dick, G. J. (2014). Microbial iron uptake as a mechanism for dispersing iron from deep-sea hydrothermal vents. Nat. Commun. 5:3192. doi: 10.1038/ncomms4192
Liang, J., Feng, J. C., Zhang, S., Cai, Y., Yang, Z., Ni, T., et al. (2021). Role of deep-sea equipment in promoting the forefront of studies on life in extreme environments. iScience 24:103299. doi: 10.1016/j.isci.2021.103299
Liang, Z. B., and Keeley, A. (2013). Filtration recovery of extracellular DNA from environmental water samples. Environ. Sci. Technol. 47, 9324–9331. doi: 10.1021/es401342b
Lloyd, K. G., Steen, A. D., Ladau, J., Yin, J., and Crosby, L. (2018). Phylogenetically novel uncultured microbial cells dominate earth microbiomes. mSystems 3, e55–e18. doi: 10.1128/mSystems.00055-18
Longnecker, K., Sievert, S. M., Sylva, S. P., Seewald, J. S., and Kujawinski, E. B. (2018). Dissolved organic carbon compounds in deep-sea hydrothermal vent fluids from the East Pacific Rise at 9°50′N. Organ. Geochem. 125, 41–49. doi: 10.1016/j.orggeochem.2018.08.004
Louca, S., Hawley, A. K., Katsev, S., Torres-Beltran, M., Bhatia, M. P., Kheirandish, S., et al. (2016). Integrating biogeochemistry with multiomic sequence information in a model oxygen minimum zone. Proc. Natl. Acad. Sci. U.S.A. 113, E5925–E5933. doi: 10.1073/pnas.1602897113
Luther, G. W., Rozan, T. F., Taillefert, M., Nuzzio, D. B., Di Meo, C., Shank, T. M., et al. (2001). Chemical speciation drives hydrothermal vent ecology. Nature 410, 813–816. doi: 10.1038/35071069
Martin, W., Baross, J., Kelley, D., and Russell, M. J. (2008). Hydrothermal vents and the origin of life. Nat. Rev. Microbiol. 6, 805–814.
Martinez, E., Vi, Dickson, J. D., Perl, S. M., and Barge, L. M. (2019). Incorporating Microbes into Synthetic Deep-Sea Hydrothermal Vents. (San Francisco, CA: American Geophysical Union, Fall Meeting).
McDermott, J. M., Sylva, S. P., Ono, S., German, C. R., and Seewald, J. S. (2018). Geochemistry of fluids from Earth’s deepest ridge-crest hot-springs: Piccard hydrothermal field, Mid-Cayman Rise. Geochimica Et Cosmochimica Acta 228, 95–118. doi: 10.1016/j.gca.2018.01.021
McNichol, J., Sylva, S. P., Thomas, F., Taylor, C. D., Sievert, S. M., and Seewald, J. S. (2016). Assessing microbial processes in deep-sea hydrothermal systems by incubation at in situ temperature and pressure. Deep-Sea Res. Part I 115, 221–232. doi: 10.1016/j.dsr.2016.06.011
McQuillan, J. S., and Robidart, J. C. (2017). Molecular-biological sensing in aquatic environments: recent developments and emerging capabilities. Curr. Opin. Biotechnol. 45, 43–50. doi: 10.1016/j.copbio.2016.11.022
Middelburg, J. J. (2018). Reviews and syntheses: to the bottom of carbon processing at the seafloor. Biogeosciences 15, 413–427. doi: 10.5194/bg-15-413-2018
Middelburg, J. J., Vlug, T., and van der Nat, F. J. W. A. (1993). Organic matter mineralization in marine systems. Glob. Planet. Change 8, 47–58. doi: 10.1016/0921-8181(93)90062-s
Mital, S., Christie, G., and Dikicioglu, D. (2021). Recombinant expression of insoluble enzymes in Escherichia coli: a systematic review of experimental design and its manufacturing implications. Microb. Cell Fact. 20:208. doi: 10.1186/s12934-021-01698-w
Miyazaki, J., Makabe, A., Matsui, Y., Ebina, N., Tsutsumi, S., Ishibashi, J., et al. (2017). WHATS-3: an improved flow-through multi-bottle fluid sampler for deep-sea geofluid research. Front. Earth Sci. 5:45. doi: 10.3389/feart.2017.00045
Moore, T. S., Mullaugh, K. M., Holyoke, R. R., Madison, A. S., Yucel, M., and Luther, G. W. (2009). Marine chemical technology and sensors for marine waters: potentials and limits. Annu. Rev. Marin. Sci. 1, 91–115. doi: 10.1146/annurev.marine.010908.163817
Mowlem, M., Beaton, A., Pascal, R., Schaap, A., Loucaides, S., Monk, S., et al. (2021). Industry partnership: lab on chip chemical sensor technology for ocean observing. Front. Marin. Sci. 8:697611. doi: 10.3389/fmars.2021.697611
Oppermann, J., Fischer, P., Silapetere, A., Liepe, B., Rodriguez-Rozada, S., Flores-Uribe, J., et al. (2019). MerMAIDs: a family of metagenomically discovered marine anion-conducting and intensely desensitizing channelrhodopsins. Nat. Commun. 10:3315. doi: 10.1038/s41467-019-11322-6
Ottesen, E. A. (2016). Probing the living ocean with ecogenomic sensors. Curr. Opin. Microbiol. 31, 132–139. doi: 10.1016/j.mib.2016.03.012
Paulus, E. (2021). Shedding light on deep-sea biodiversity - a highly vulnerable habitat in the face of anthropogenic change. Front. Marin. Sci. 8:667048. doi: 10.3389/fmars.2021.667048
Perner, M., Gonnella, G., Hourdez, S., Böhnke, S., Kurtz, S., and Girguis, P. (2013). In situ chemistry and microbial community compositions in five deep-sea hydrothermal fluid samples from Irina II in the Logatchev field. Environ. Microbiol. 15, 1551–1560. doi: 10.1111/1462-2920.12038
Perner, M., Gonnella, G., Kurtz, S., and LaRoche, J. (2014). Handling temperature bursts reaching 464°C: different microbial strategies in the Sisters Peak hydrothermal chimney. Appl. Environ. Microbiol. 80, 4585–4598. doi: 10.1128/AEM.01460-14
Perner, M., Ilmberger, N., Köhler, H. U., Chow, J., and Streit, W. R. (2011b). “Emerging fields in functional metagenomics and its industrial relevance: overcoming limitations and redirecting the search for novel biocatalysts,” in Handbook of Moleculare Microbial Ecology II, ed. F. J. De Bruijn (New Jersey, NJ: Wiley-Blackwell), 484–485.
Perner, M., Hentscher, M., Rychlik, N., Seifert, R., Strauss, H., and Bach, W. (2011a). Driving forces behind the biotope structures in two low-temperature hydrothermal venting sites on the southern Mid-Atlantic Ridge. Environ. Microbiol. Rep. 3, 727–737. doi: 10.1111/j.1758-2229.2011.00291.x
Petersen, J. M., Zielinski, F. U., Pape, T., Seifert, R., Moraru, C., Amann, R., et al. (2011). Hydrogen is an energy source for hydrothermal vent symbioses. Nature 476, 176–180. doi: 10.1038/nature10325
Pillot, G., Frouin, E., Pasero, E., Godfroy, A., Combet-Blanc, Y., Davidson, S., et al. (2018). Specific enrichment of hyperthermophilic electroactive Archaea from deep-sea hydrothermal vent on electrically conductive support. Biores. Technol. 259, 304–311. doi: 10.1016/j.biortech.2018.03.053
Pushkarev, A., Inoue, K., Larom, S., Flores-Uribe, J., Singh, M., Konno, M., et al. (2018). A distinct abundant group of microbial rhodopsins discovered using functional metagenomics. Nature 558, 595–599. doi: 10.1038/s41586-018-0225-9
Rabausch, U., Jürgensen, J., Ilmberger, N., Böhnke, S., Fischer, S., Schubach, B., et al. (2013). Functional screening of metagenome and genome libraries for detection of novel flavonoid-modifying enzymes. Appl. Environ. Microbiol. 79, 4551–4563. doi: 10.1128/AEM.01077-13
Reed, D. C., Algar, C. K., Huber, J. A., and Dick, G. J. (2014). Gene-centric approach to integrating environmental genomics and biogeochemical models. Proc. Natl. Acad. Sci. U.S.A. 111, 1879–1884. doi: 10.1073/pnas.1313713111
Resing, J. A., Sedwick, P. N., German, C. R., Jenkins, W. J., Moffett, J. W., Sohst, B. M., et al. (2015). Basin-scale transport of hydrothermal dissolved metals across the South Pacific Ocean. Nature 523, 200–203. doi: 10.1038/nature14577
Reysenbach, A.-L., and Götz, D. (2001). Methods for the study of hydrothermal vent microbes. Methods Microbiol. 30, 639–656. doi: 10.1016/s0580-9517(01)30066-1
Rinke, C., Schwientek, P., Sczyrba, A., Ivanova, N. N., Anderson, I. J., Cheng, J. F., et al. (2013). Insights into the phylogeny and coding potential of microbial dark matter. Nature 499, 431–437. doi: 10.1038/nature12352
Robidart, J., Callister, S. J., Song, P. F., Nicora, C. D., Wheat, C. G., and Girguis, P. R. (2013). Characterizing Microbial Community and Geochemical Dynamics at Hydrothermal Vents Using Osmotically Driven Continuous Fluid Samplers. Environ. Sci. Technol. 47, 4399–4407. doi: 10.1021/es3037302
Sanchez, A. M. (2021). Exploring habitability with artificial hydrothermal vents. Nat. Rev. Earth Environ. 2:590. doi: 10.1038/s43017-021-00206-3
Sander, S. G., and Koschinsky, A. (2011). Metal flux from hydrothermal vents increased by organic complexation. Nat. Geosci. 4, 145–150. doi: 10.1038/ngeo1088
Sass, K., Güllert, S., Streit, W. R., and Perner, M. (2020). A hydrogen-oxidizing bacterium enriched from the open ocean resembling a symbiont. Environ. Microbiol. Rep. 12, 396–405. doi: 10.1111/1758-2229.12847
Sass, K., and Perner, M. (2020). Characterization of two hydrogen-oxidizing Hydrogenovibrio strains from Kermadec Volcanic Island Arc hydrothermal vents. Front. Marin. Sci. 7:295. doi: 10.3389/fmars.2020.00295
Scholin, C. A., Birch, J., Jensen, S., Marin, R., Massion, E., Pargett, D., et al. (2017). The quest to develop ecogenomic sensores - a 25-year history of the Environmental Sample Processor (ESP) as a case study. Oceanography 30, 100–113. doi: 10.5670/oceanog.2017.427
Seewald, J. S., Doherty, K. W., Hammar, T. R., and Liberatore, S. P. (2002). A new gas-tight isobaric sampler for hydrothermal fluids. Deep-Sea Res. Part I 49, 189–196. doi: 10.1016/s0967-0637(01)00046-2
Shakya, M., Lo, C. C., and Chain, P. S. G. (2019). Advances and challenges in metatranscriptomic analysis. Front. Gen. 10:904. doi: 10.3389/fgene.2019.00904
Sievert, S. M., and Vetriani, C. (2012). Chemoautotrophy at deep-sea vents: past, present, and future. Oceanography 25, 218–233. doi: 10.5670/oceanog.2012.21
Simon, C., and Daniel, R. (2011). Metagenomic analyses: past and future trends. Appl. Environ. Microbiol. 77, 1153–1161. doi: 10.1128/AEM.02345-10
Somes, C. J., Dale, A. W., Wallmann, K., Scholz, F., Yao, W. X., Oschlies, A., et al. (2021). Constraining global marine iron sources and ligand-mediated scavenging Fluxes With GEOTRACES Dissolved Iron Measurements in an Ocean Biogeochemical Model. Glob. Biogeochem. Cycles 35:e2021GB006948.
Stokke, R., Reeves, E. P., Dahle, H., Fedøy, A. E., Viflot, T., Lie Onstad, S., et al. (2020). Tailoring hydrothermal vent biodiversity toward improved biodiscovery using a novel In situ enrichment strategy. Front. Microbiol. 11:249. doi: 10.3389/fmicb.2020.00249
Sunagawa, S., Coelho, L. P., Chaffron, S., Kultima, J. R., Labadie, K., Salazar, G., et al. (2015). Ocean plankton. Structure and function of the global ocean microbiome. Science 348:1261359. doi: 10.1126/science.1261359
Svetlitsky, D., Dagan, T., and Ziv-Ukelson, M. (2020). Discovery of multi-operon colinear syntenic blocks in microbial genomes. Bioinformatics 36, 21–29. doi: 10.1093/bioinformatics/btaa503
Taylor, C. D., Edgcomb, V. P., Doherty, K. W., Engstrom, I., Shanahan, T., Pachiadaki, M. G., et al. (2015). Fixation filter, device for the rapid In situ preservation of particulate samples. Deep Sea Res. Part I 96, 69–79. doi: 10.1016/j.dsr.2014.09.006
Thoms, F., Burmeister, C., Dippner, J. W., Gogina, M., Janas, U., Kendzierska, H., et al. (2018). Impact of macrofaunal communities on the coastal filter function in the Bay of Gdansk, Baltic Sea. Front. Marin. Sci. 5:201. doi: 10.3389/fmars.2018.00201
Toner, B. M., Fakra, S. C., Manganini, S. J., Santelli, C. M., Marcus, M. A., Moffett, J., et al. (2009). Preservation of iron(II) by carbon-rich matrices in a hydrothermal plume. Nat. Geosci. 2, 197–201. doi: 10.1038/ngeo433
Varaljay, V. A., Satagopan, S., North, J. A., Witte, B., Dourado, M. N., Anantharaman, K., et al. (2016). Functional metagenomic selection of RubisCO from uncultivated bacteria. Environ. Microbiol. 18, 1187–1199. doi: 10.1111/1462-2920.13138
Venter, J. C., Remington, K., Heidelberg, J. F., Halpern, A. L., Rusch, D., Eisen, J. A., et al. (2004). Environmental genome shotgun sequencing of the Sargasso Sea. Science 304, 66–74. doi: 10.1126/science.1093857
Vonnahme, T. R., Molari, M., Janssen, F., Wenzhofer, F., Haeckel, M., Titschack, J., et al. (2020). Effects of a deep-sea mining experiment on seafloor microbial communities and functions after 26 years. Sci. Adv. 6:eaaz5922.
Wang, S., Wu, S. J., Du, M. R., Yang, C. J., and Wang, X. (2020). A new serial sampler for collecting gas-tight samples from seafloor cold seeps and hydrothermal vents. Deep Sea Res. Part I 161:103282. doi: 10.1016/j.dsr.2020.103282
Wankel, S. D., Germanovich, L. N., Lilley, M. D., Genc, G., DiPerna, C. J., Bradley, A. S., et al. (2011). Influence of subsurface biosphere on geochemical fluxes from diffuse hydrothermal fluids. Nat. Geosci. 4, 461–468. doi: 10.1038/ngeo1183
Wilmes, P., Heintz-Buschart, A., and Bond, P. L. (2015). A decade of metaproteomics: where we stand and what the future holds. Proteomics 15, 3409–3417. doi: 10.1002/pmic.201500183
Wu, S. J., Wang, S., and Yang, C. J. (2018). Collection of gas-tight water samples from the bottom of the challenger deep. J. Atmos. Ocean. Technol. 35, 837–844. doi: 10.1175/jtech-d-17-0170.1
Yang, C., Xia, Y., Qu, H., Li, A. D., Liu, R., Wang, Y., et al. (2016). Discovery of new cellulases from the metagenome by a metagenomics-guided strategy. Biotechnol. Biofuels 9:138. doi: 10.1186/s13068-016-0557-3
Zamkovaya, T., Foster, J. S., de Crécy-Lagard, V., and Conesa, A. (2021). A network approach to elucidate and prioritize microbial dark matter in microbial communities. ISME J. 15, 228–244. doi: 10.1038/s41396-020-00777-x
Zeng, X., Alain, K., and Shao, Z. (2021). Microorganisms from deep-sea hydrothermal vents. Marin. Life Sci. Technol. 3, 204–230. doi: 10.1007/s42995-020-00086-4
Zeng, X., Zhang, X., Jiang, L., Alain, K., Jebbar, M., and Shao, Z. (2013). Palaeococcus pacificus sp. nov., an archaeon from deep-sea hydrothermal sediment. Int. J. Syst. Evol. Microbiol. 63, 2155–2159. doi: 10.1099/ijs.0.044487-0
Keywords: hydrothermal vents, uncultured microbial majority, microbial dark matter, functional metagenomics, in situ technologies, activity-based screening, novel enzymes
Citation: Böhnke S and Perner M (2022) Approaches to Unmask Functioning of the Uncultured Microbial Majority From Extreme Habitats on the Seafloor. Front. Microbiol. 13:845562. doi: 10.3389/fmicb.2022.845562
Received: 30 December 2021; Accepted: 07 March 2022;
Published: 29 March 2022.
Edited by:
Andreas Teske, University of North Carolina at Chapel Hill, United StatesReviewed by:
Ida Helene Steen, University of Bergen, NorwayJason B. Sylvan, Texas A&M University, United States
Copyright © 2022 Böhnke and Perner. This is an open-access article distributed under the terms of the Creative Commons Attribution License (CC BY). The use, distribution or reproduction in other forums is permitted, provided the original author(s) and the copyright owner(s) are credited and that the original publication in this journal is cited, in accordance with accepted academic practice. No use, distribution or reproduction is permitted which does not comply with these terms.
*Correspondence: Stefanie Böhnke, sboehnke-brandt@geomar.de; Mirjam Perner, mperner@geomar.de