Environmental and physiological conditions that led to the rise of calcifying nannoplankton in the Late Triassic
- 1Leibniz Centre for Tropical Marine Research (ZMT), Department of Integrated Modelling, Bremen, Germany
- 2State Key Laboratory of Biogeology and Environmental Geology, China University of Geosciences, Wuhan, China
- 3Department of Biology, Colorado State University, Fort Collins, CO, United States
- 4Division of Biosciences, Alfred Wegener Institute for Polar and Marine Research (AWI), Bremerhaven, Germany
- 5School of Science, Constructor University, Bremen, Germany
The rise of calcifying nannoplankton in the Late Triassic (237–201 Ma) had profound consequences for the carbonate buffering capacity of the ocean. Research on the appearance of calcification typically focuses on the reasons that made this process successful on a global scale. The underlying environmental and physiological conditions that led to its appearance, therefore, are still obscure. Here we show that the rise of intracellular calcification is intrinsically linked to cellular calcium metabolism and to the prevention of calcium accumulation in the cytoplasm. Using gene tree analysis, we present evidence that the physiological machinery for calcification was already present in non-calcifying ancestor cells. Additionally, by modelling the energy demands for calcium transport in calcifying and non-calcifying cells, we demonstrate that intracellular calcification does not require additional energy investments. Since all eukaryotic cells export calcium across the plasma membrane, our findings indicate that the onset of intracellular calcification in Earth’s history required only the activation of calcium transport proteins during their passage to the plasma membrane. Hence, intracellular calcification could have occurred at any time in the geological past because physiological preconditions were already present, but a combination of favourable environmental factors may have helped calcifying nannoplankton to spread at the beginning of the Mesozoic, a time of catastrophic climate changes and mass extinctions that reshuffled life on Earth.
1 Introduction
Pelagic nannoplankton calcifiers appeared in the Late Triassic (Bralower et al., 1991; Bown, 1998; Bown et al., 2004; Onoue and Yoshida, 2010; Gardin et al., 2012; Preto et al., 2013b; a), Figure 1. The first were probably small calcareous dinoflagellates that produced simple calcispheres (on average about 10 μm in diameter) composed of sub-micron scale calcium carbonate crystals, which appeared during the Carnian, about 233 Ma, when modern marine fauna was rising in the aftermath of the most catastrophic mass extinction event of the entire Phanerozoic Eon at the Permian-Triassic boundary (Janofske, 1992; Onoue and Yoshida, 2010; Preto et al., 2013b; Preto et al., 2013a; Dal Corso et al., 2021), Figure 1. Coccolithophores, which are haptophyte algae and secrete complex ornamented calcite plates (Young, 2003; Young et al., 2005), appeared in the middle-late Norian, about 215–210 Ma (Gardin et al., 2012; Demangel et al., 2020), and spread after the Triassic-Jurassic mass extinction event (e.g.,: Bown et al., 2004), Figure 1. Before the emergence of pelagic nannoplankton calcification, the biological precipitation of calcium carbonate was dominated by metazoans and microbial mats that calcified extracellularly in a medium isolated from seawater (Lowenstam and Margulis, 1980; Kaźmierczak et al., 1985; Knoll, 2003). In contrast, calcareous nannoplankton, such as calcareous dinoflagellates (calcispheres) and coccolithophores, calcify inside intracellular compartments called vesicles and extrude the accreted crystals via exocytosis (Inouye and Pienaar, 1983; Paasche, 2002; Brownlee et al., 2015; Jantschke et al., 2020). The emergence of intracellular calcification allowed biocalcification to expand into the surface waters of the open ocean, marking the transition from the so-called “Neritan” ocean to the “Cretan” ocean (Zeebe and Westbroek, 2003; Ridgwell, 2005; de Vargas et al., 2007), Figure 1. This expansion produced a much more efficient regulation of the global carbon cycle and changed the marine calcium carbonate saturation state, transforming the entire ocean carbonate chemistry (Zeebe and Westbroek, 2003; Ridgwell, 2005; Ridgwell and Zeebe, 2005). The production of intracellular platelets that are then extruded onto the outer cell surface led to a completely new phenotype with respect to non-calcifying nannoplankton. The emergence of pelagic calcareous nannoplankton is, thus, one of the most important evolutionary innovations in Earth’s history but how and why it originated is still unclear. The mechanisms driving evolutionary innovations are mutation and selection. Therefore, before identifying the benefits that could have selected intracellular calcification to make it a successful innovation at a global scale, it is important to understand the physiological characteristics (or mutations) required for its emergence.
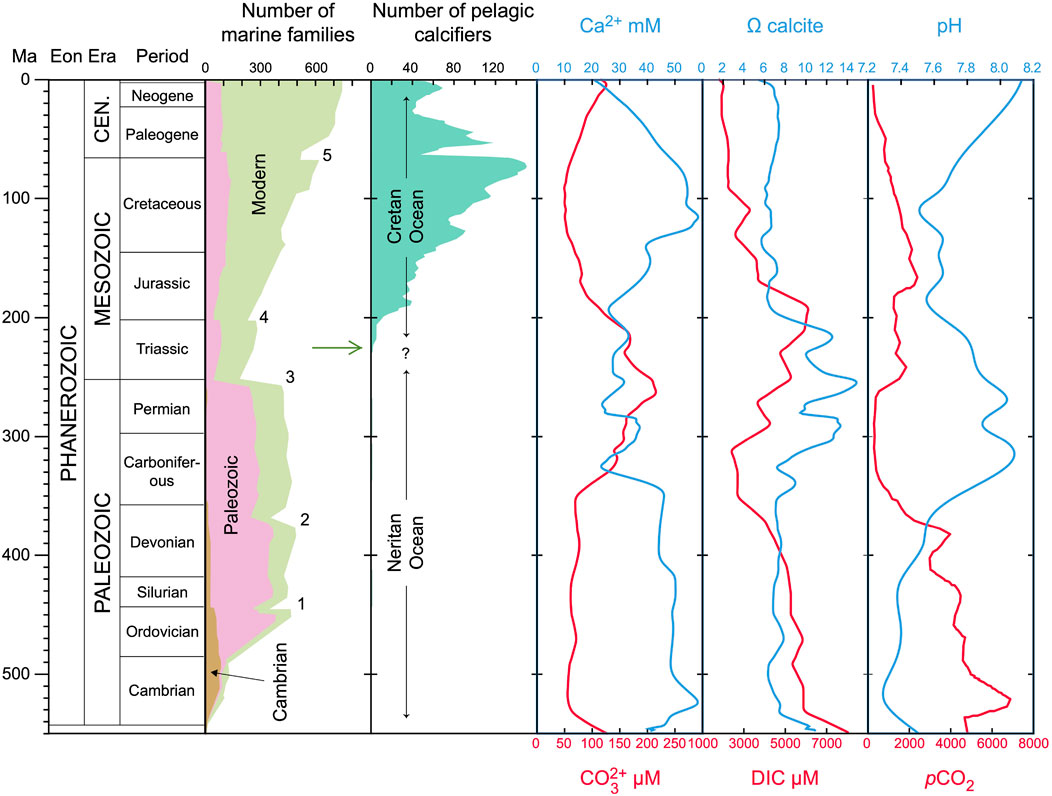
FIGURE 1. Fossil record of pelagic calcifiers and paleoceanographic carbonate chemistry over the past 550 Myr. The number of marine families and evolutionary faunas throughout the Phanerozoic are from Raup and Sepkoski Jr (1982) and Sepkoski and Ausich (1990). The numbers indicate the 5 major mass extinction events (1 = Ordovician–Silurian Extinction; 2 = Late Devonian Extinction; 3 = Permian–Triassic Extinction; 4 = Triassic–Jurassic Extinction; 5 = Cretaceous–Paleogene Extinction). The number of pelagic nannoplankton calcifiers is reconstructed by Bown et al. (2004). Note, however, that the data in Bown et al. (2004) include pelagic calcifiers in general and not exclusively nannoplankton. The concentration of calcium is taken from Stanley and Hardie (1998) and atmospheric pCO2 from Berner and Kothavala (2001). Dissolved inorganic carbon (DIC) and ocean pH were reconstructed by Ridgwell (2005). The calcite saturation state (Ωcalcite) was calculated, using the standard software CO2sys (Lewis and Wallace, 1998), from Ca2+ and
While the evolution of extracellular calcification was likely an adaptive response to rising calcium concentrations in the ocean (Kaźmierczak et al., 1985; Kaźmierczak and Kempe, 2004), the appearance of intracellular calcification does not clearly correlate with changes in ocean chemistry (Figure 1). For example, a temporal coincidence between a Late Triassic drastic drop in atmospheric pCO2, resulting from global carbon-climate numerical modelling, and the primary radiation of calcareous nannoplankton, inferred from the fossil record, has been observed but a cause-and-effect link between the two events could not be proven (Goddéris et al., 2008).
Moreover, when intracellular calcification emerged, the concentration of calcium in the ocean was approximately 30 mM, dissolved inorganic carbon (DIC) was 5,000 μM, and ocean pH was about 7.8 (Figure 1). These conditions would have led to a carbonate concentration of 160 μM and a calcite saturation state of 10. Although such chemical conditions would be favourable to calcification and could therefore positively select for this innovation, they alone are not sufficient to explain the appearance of intracellular calcification because the ocean experienced similar conditions 80–100 million years before the first evidence of pelagic nannoplankton calcification appears in the geological record (Late Triassic-Jurassic). The ocean experienced even higher calcium concentrations (from 50 to almost 60 mM) from the Cambrian to the Carboniferous and calcite saturation reached almost 15 in the Carboniferous and Permian. Therefore, it is plausible to assume that the emergence of intracellular calcification was most likely a consequence of random changes in cellular physiology rather than a response to external environmental factors such as changes in ocean carbonate chemistry. Using new energy budget calculations and gene analyses, we investigate here the underlying mechanisms and prerequisites that allowed intracellular calcification to appear. More explicitly, we investigate if the proteins and ion transporters involved in intracellular calcification were present in non-calcifying ancestors or appeared coincidentally with the emergence of intracellular calcification. Furthermore, we explore if intracellular calcification is indeed energetically costly as proposed by Monteiro et al. (2016) so that secondary evolutionary benefits must compensate for the energy invested into calcification. This is done by calculating the amounts of energy required to pump calcium ions into the calcifying vesicles in comparison to maintaining calcium homeostasis in all (calcifying and non-calcifying) cells.
2 Materials and methods
2.1 Analysis of environmental data
We calculated the carbonate chemistry parameters from reconstructed historic ocean pH and DIC (Ridgwell, 2005) using the standard software CO2sys, version 1.01 (Lewis and Wallace, 1998). Calculated ocean carbonate concentrations and reconstructed calcium concentrations (Stanley and Hardie, 1998) were used to determine the calcite saturation state. Atmospheric pCO2 was obtained from previous reconstructions (Berner and Kothavala, 2001). We compared the carbonate chemistry parameters with the fossil record of pelagic calcifying nannoplankton (Bown et al., 2004) to identify the environmental conditions under which intracellular calcification emerged (Figure 1). The primary objective of this analysis is to compare, in qualitative terms, the reconstructed carbonate chemistry of the ocean with the appearance of calcareous nannoplankton. A precise, quantitative reconstruction of the carbonate chemistry throughout the Phanerozoic goes beyond the scope of our study. However, from a visual inspection, the data we compiled using literature sources and CO2sys are consistent with recent reconstructions (Witkowski et al., 2018; Montañez et al., 2016).
2.2 Calcium-transporter gene tree analysis
To examine the evolutionary history of calcification-related proteins, we identified proteins across lineages and created gene trees of relevant protein families. We downloaded protein databases from the National Center for Biotechnology Information (NCBI) genome browser for taxa represented in the phylogeny of Read et al. (2013), Supplementary Table S1. To account for differences in annotation completeness in each protein database, we used BLAST to identify homologs of Gephyrocapsa huxleyi proteins in each taxon. We searched the G. huxleyi protein database for annotated sequences in each protein family implicated in calcification (Benner et al., 2013) to use as query sequences (Supplementary Table S2). We then used pBLAST to identify all sequences in the protein database that matched these query sequences with probabilities less than e-6. After discarding duplicates, we aligned the BLAST results for each protein family in Geneious 11.1.3, (Kearse et al., 2012), http://www.geneious.com, using the Geneious global alignment with cost matrix Blosum62, gap open penalty 12, and gap extension penalty 3. We created gene trees using Jukes-Cantor genetic distance module and the UPGMA tree building method in Geneious. We then inspected trees for phylogenetic representation and patterns of protein family expansion. Because the BLAST output results for calcium channels included both cation channels and calmodulin, we split these sequences into two subtrees for better visualization of the relationships among calmodulin-related proteins. Supplementary Figures S1 – S7 show the resulting gene trees.
2.3 Calculation of energy invested into intracellular calcification
We calculated the energy required for calcium transport into Golgi-derived vesicles as part of the energy invested to maintain calcium homeostasis. In our calculations, we neglect the costs associated to the transport of golgi vesicles because these vesicles are transported by both calcifying and non-calcifying cells. We also neglect the costs required for the production of polysaccharides and for exocytosis, the process for the secretion of polysaccharides by haptophytes, because also these costs are inccurred by non-calcifying cells and, thus, do not constitute and additional energy requirement for calcifying cells. In order to achieve intracellular calcium homeostasis, total calcium transport out of the cytoplasm has to equal the passive influx via calcium channels. The passive, diffusive uptake via the calcium channels is driven by the difference between the calcium concentration at the cell surface (Casurf) and the calcium concentration in the cytoplasm (Cacyt). Thus, knowing the calcium gradient across the plasma membrane, the energy required to achieve calcium homeostasis (EATP) can be calculated by
where PCa is the apparent membrane permeability per cell surface area (Acell) and
where DCa is the diffusion coefficient of calcium in seawater and rcell is the cell radius. Here, we assume that the transport of calcium into vesicles represents a fixed fraction of total calcium transport (fv) as compared to the transport over the plasma membrane (1 − fv), i.e.,:
In other words, we assume that while calcium is rushing in through the ion channels, ATPases pump calcium back through the cell membrane into the extracellular medium but at the same time also into the vesicles. The volume inside the vesicle, however, can be regarded as outside of the cytoplasm from a thermodynamical point of view. In order to maintain calcium homeostasis, the flux into the cytoplasm has to be balanced by the fluxes out of the cytoplasm. Inserting the expression for Qvesicle into Eq. 2 and subtracting Cacyt from both sides of Eq. 2 results in an implicit equation for the concentration difference Casurf - Cacyt, which has the solution:
Replacing in Eq. 1 the concentration difference Casurf − Cacyt with Eq. 4 gives EATP as a function of the relative fraction of calcium transport into the calcifying vesicles:
This equation shows that the higher fv, the lower the absolute requirements for cellular energy. In order to quantify how much the cellular energy consumption can potentially be reduced by intracellular calcification, we calculate the energy requirements to maintain calcium homeostasis as a function of fv from 0 (non-calcifying cell) to 1 (Supplementary Figure S8). The coccolithophore Gephyrocapsa huxleyi produces approximately 1 coccolith per hour (Paasche, 1962) and one coccolith contains around 22 fmol of CaCO3 (Young and Ziveri, 2000). This results in a calcification flux (Qvesicle) of about 6.11 ⋅ 10–18 mol s−1 (see: Holtz et al., 2013). Since the permeability of the plasma membrane for calcium ions is not known in coccolithophores, we estimate a permeability that is based on the conductance of calcium channels (Lux and Nagy, 1981) and measured densities of calcium channels in cell membranes of snail axons (Krishtal et al., 1981; Tsien, 1983). All the parameter values used to calculate the extra energy required by intracellular calcification are reported in Supplementary Table S3. We provide the numerical code for calculating the energy required to maintain calcium homeostasis by single-celled calcifying nannoplankton as open-source software (https://github.com/systemsecologygroup/Calcisphere) so that it can be used, modified, and redistributed freely.
2.4 Calculation of intracellular calcium poisoning
The energy for the Ca2+ pumping ATPase is provided by the hydrolysis of ATP at a high ATP/ADPxPi ratio in the cytosol with a free energy gain, ΔG, of 15 kcal/mol (Meldolesi and Pozzan, 1998). Cooperative binding of 2 Ca2+ per ATPase implies a Ca2+/ATP coupling ratio of 2 (Inesi and Tadini-Buoninsegni, 2014). We assume an electroneutral Ca2+/2H+ co-transport for the Ca2+ pump and a physiological pH at both sides of the Ca2+ pump. If all of the free energy available from ATP hydrolysis is stored in the concentration gradient of Ca2+ between the cytosol and its environment, i.e.,: ΔG = 2RT ⋅ ln (Casurf/Cacyt), the calcium gradient can be up to 105. If the Ca-ATPases work near this thermodynamic limit, the cytosolic Ca2+ concentration of 0.1 μmol L−1 and the gradient of 105 yields a Ca2+ concentration of 10 mM at the cell surface, which is, in fact, exactly the Ca2+ concentration in today’s ocean (Langer et al., 2006). Ca2+ transport near the thermodynamic limit would have the advantages that: 1) pumping Ca2+ from the cytosol to the environment proceeds with a minimum dissipation of energy in ATP consumption, and 2) a steep Ca2+ gradient provides fast influx in cell signaling. We therefore postulate that a constant Ca2+-gradient close to the thermodynamic limit represents a part of calcium homeostasis. Moreover, the Ca2+-gradient across the plasma membrane should be quite independent of the bulk Ca2+ concentration in seawater.
Assuming that the Ca2+-gradient across the plasma membrane is maintained at 105 by the Ca2+-ATPase, the intracellular calcium concentration will respond to variations in extracellular calcium concentration as experienced during Earth’s history (Stanley and Hardie, 1998), Figure 1. The intracellular concentration of calcium (Cacyt), however, generates a constraint on the intracellular phosphate concentration (POcyt) because calcium and phosphate precipitate to Ca3(PO4)2 when the ion product exceeds the solubility product
3 Results and discussion
3.1 Calcium metabolism
Calcium ions play important roles in the physiology of eukaryotic cells, including control of DNA synthesis, chromosomal configuration, and signal transduction (Marcum et al., 1978; Boynton et al., 1980; Krishtal et al., 1981; Lux and Nagy, 1981; Tsien, 1983; Poenie et al., 1985; Carafoli, 1987; Steinhardt and Alderton, 1988; Hepler, 1994; Sanders et al., 2002; Verret et al., 2010). Calcium ions enter the cell passively via channels (Gussone et al., 2006; Verret et al., 2010) that allow for a diffusive influx driven by a steep concentration gradient across the plasma membrane (Langer et al., 2006). Excess intracellular Ca2+ is then actively removed by calcium-binding proteins (e.g., calmodulin) and transport proteins (Ca2+-ATPase and Na+/Ca2+ exchanger) with different calcium affinities and maximum transport rates (Carafoli, 1987). The Ca2+ concentration inside the cell has to be tightly regulated (calcium homeostasis) at around 0.1 μmol L−1 (Brownlee et al., 1995) for optimal cell functioning (Carafoli, 1987) and to avoid cell poisoning (Simkiss, 1977; Orrenius et al., 1989; Müller et al., 2015).
The same proteins that maintain intracellular calcium homeostasis in non-calcifying cells (Figure 2A) are implicated in intracellular calcification (Figure 2B). To determine whether an expansion of protein families coincides with the emergence of intracellular calcification, we compared all gene families involved in the precipitation of calcium carbonate (von Dassow et al., 2009) in a large number of eukaryotes (Read et al., 2013). These genes include Ca2+ transporting ATPases,
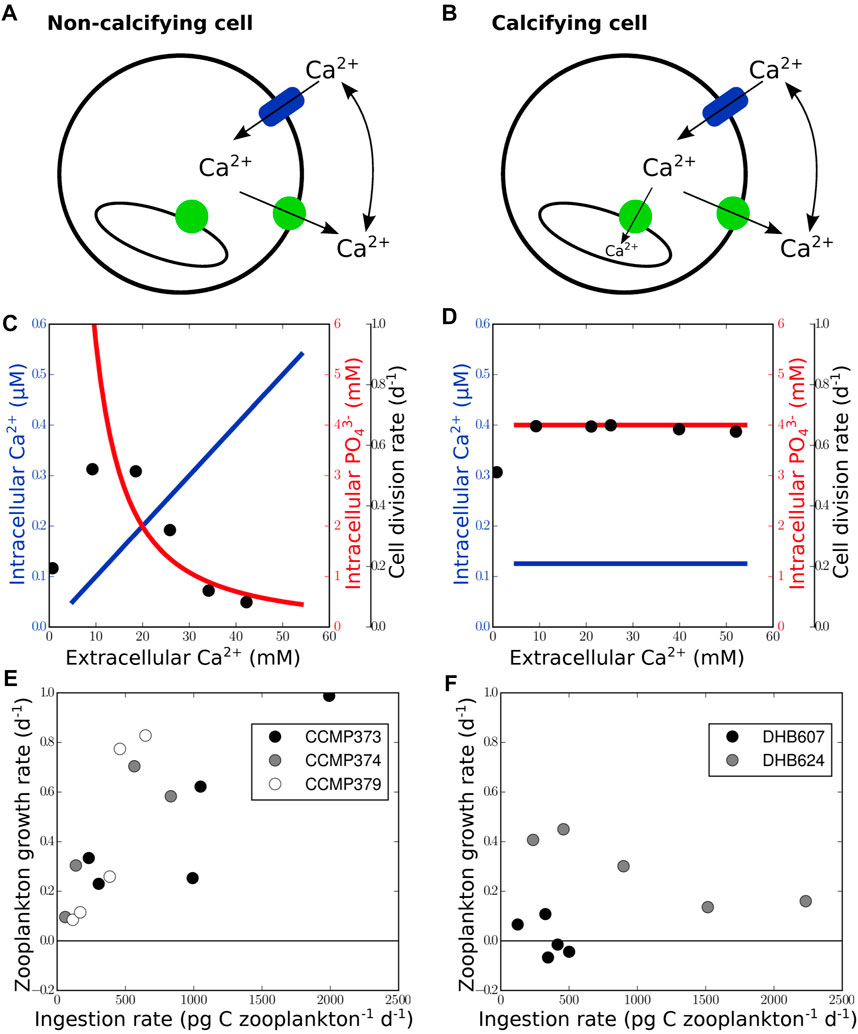
FIGURE 2. Physiological and ecological benefits of intracellular calcification. Calcifying cells (B) differ from non-calcifying cells (A) by the presence of intravesicular calcium accumulation in Golgi-derived transport vesicles (green circles represent Ca2+ pump/ATPase; blue cylinder indicates calcium channel). Calcium transport into vesicles allows maintenance of calcium homeostasis under elevated extracellular calcium concentrations (D) and prevents drawdown of intracellular phosphate (C) due to precipitation of Ca3(PO4)2 in the cytosol. Therefore, cell division rates, black dots in (C) and (D), redrawn from Müller et al. (2015), remain unaffected by extracellular calcium concentrations in calcifying cells contrary to non-calcifying cells. The growth rate of zooplankton fed with calcifying cells (F) is generally lower than that of zooplankton fed with non-calcifying cells (E), data taken from Harvey et al. (2015) and originally produced from batch cultures in exponential growth phase by Strom et al. (2003).
The most significant difference between calcifying and non-calcifying cells is that calcium carbonate precipitates inside Golgi-derived vesicles. The vesicles fuse with the plasma membrane and the calcium carbonate is extruded outside the cell where it remains attached to the extracellular organic matrix. The same vesicular pathway is used in non-calcifying cells to produce new membrane material and to transport proteins to the plasma membrane (Figure 3). All ion transporters and channels that help establish calcium homeostasis in non-calcifying cells, therefore, reach the plasma membrane via Golgi-derived transport vesicles (Pfeffer and Rothmann, 1987). However, the Ca2+ transporting ATPase initially occurs in its auto-inhibited form and becomes activated by the presence of calmodulin (Tidow et al., 2012) at the plasma membrane. We thus argue that the emergence of intracellular calcification was triggered by the activation of calcium pumps during the passage of transport vesicles from the Golgi apparatus to the plasma membrane, following the accumulation of calcium in the transport vesicles. This explanation is consistent with the simple structure of sub-micron scaled calcite crystals of proto-calcispheres found in previous studies and attributed to calcareous dinoflagellates (Janofske, 1992; Preto et al., 2013b; Dal Corso et al., 2021). The activation of the calcium transport inside the Golgi apparatus would later lead to the formation of more elaborated coccolith-like platelets due to the specific endomembranous structure of the Golgi apparatus (Figure 3). This is also in line with the finding that inhibition of the cytoskeleton and microtubule formation induces malformations in modern coccolith (Langer et al., 2010).
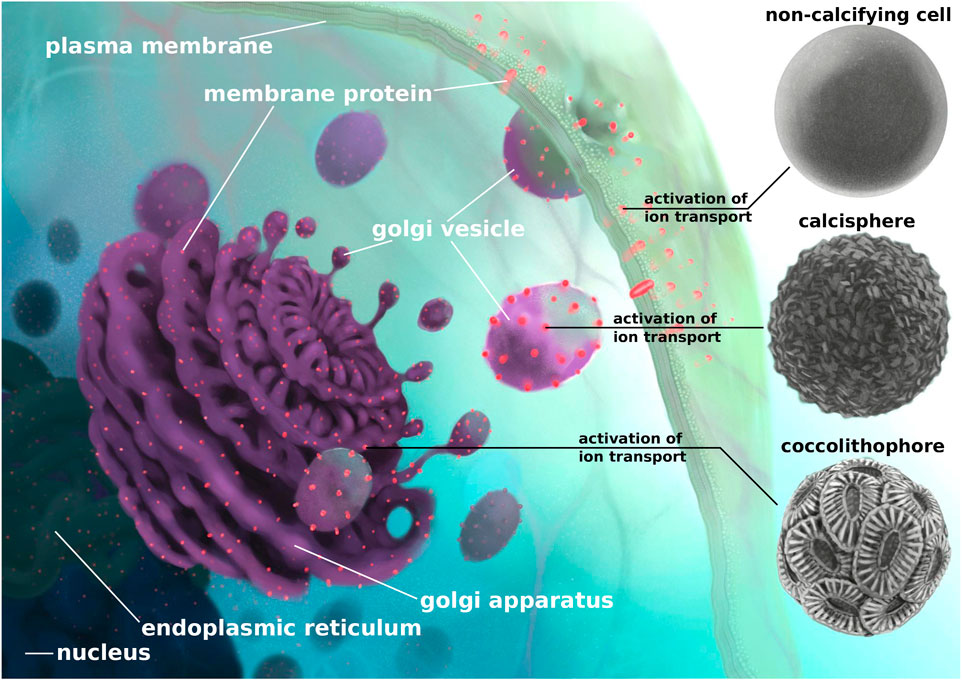
FIGURE 3. Schematic reconstruction of the steps that, based on our findings, would have led to the emergence of nannoplankton calcification. After transcription from DNA to mRNA in the nucleus, gene copies are translated into proteins in the endoplasmic reticulum. Proteins that are bound to the plasma membrane are incorporated into the ER membrane and are transported via vesicles to the Golgi apparatus. Golgi-derived vesicles are then transported to the plasma membrane where the vesicles fuse and shed their content to the extracellular space. The vesicle membrane, including all membrane proteins, becomes part of the plasma membrane. We suggest that, in a non-calcifying cell, the activation of calcium transporters occurs at the plasma membrane. Earlier activation of calcium transporters in transport vesicles would allow precipitation of sub-micron scale crystals inside the vesicles that could have led to the formation of proto-calcispheres by calcareous dinoflagellates (Janofske, 1992; Preto et al., 2013b; Dal Corso et al., 2021). The activation of calcium transporters, in the more structurally complex endomembranous systems like the Golgi apparatus, could explain the formation of delicate coccoliths and the emergence of modern-like coccolithophores. The chronological order of the appearance of calcispheres and coccolithophores shown in the diagram (from top to bottom) does not necessarily represent direct evolutionary steps regarding the activation of calcium transporters but may represent parallel origins of early Ca2+ transport activation that happened independently (Meier et al., 2007).
Such a change in timing of activation would not be detected in gene sequence comparison, as they require protein functional assays. We conclude, however, that all components necessary for the emergence of intracellular calcification were already present in non-calcifying ancestor cells and that no major modifications of cellular physiology and structure were required to induce intracellular calcification. An alternative and speculative hypothesis to the activation of calcium pumps could be that those pumps were already active and that the precipitation of calcium carbonate in the vesicles was prevented by inhibitors, which were subsequently lost thus leading to the emergence of intracellular calcification. But also in this alternative case, the emergence of intracellular calcification would not require major innovations in cellular structure, which is our main argument here.
3.2 Energy requirements
Since ion transport requires energy, a previous study (Monteiro et al., 2016) assumed that intracellular calcification is energetically costly, thus implying the presence of associated benefits to overcome natural selection. But because non-calcifying cells also export calcium from their cytoplasm, the energetic costs of calcification have to be quantified by comparing the costs required for ion transport into vesicles in calcifying cells versus those required by ion transport across the plasma membrane (to maintain calcium homeostasis) in both calcifying and non-calcifying cells. We therefore assessed the energetic costs of intracellular calcification by calculating the total energy required by cellular calcium transport to maintain calcium homeostasis. Our calculations indicate that the precipitation of CaCO3 inside the vesicle removes calcium from the solution and generates a sink that depletes calcium around the cell, Eq. 2. Consequently, the gradient across the plasma membrane that drives the diffusive calcium influx through the channel is reduced with respect to non-calcifying cells, Eq. 4, thus fewer ions have to be transported out of the cytoplasm in order to maintain calcium homeostasis, Eq. 5. Since the energy required for active calcium transport out of a calcifying cell is negligibly lower than that required in non-calcifying cells (Supplementary Figure S8), we conclude that extra energy is not needed to transport calcium for intracellular calcification.
However, our energy calculations also show that the reduction in energy requirements is very small because the calcification flux is slow compared to extracellular calcium diffusion so that no significant diffusive boundary layer develops at the cell surface. If all calcium ions were transported into the vesicles and no calcium were exported through the plasma membrane, fv = 1.0 in Eq. 5, the energy requirements would only be 0.16 percent less than if no calcium were transported into vesicles and all ions were exported through the plasma membrane, fv = 0.0 in Eq. 5.
Besides calcium ions, also carbonate ions are needed in the vesicle to sustain calcification. The transport of carbon could thus be another process requiring energy. However, the transport of hydrogen ions that is coupled to calcium transport creates an alkaline medium inside the calcifying vesicle that shifts the equilibrium of the carbonate system towards carbonate ions and lowers the CO2 concentration (Holtz et al., 2013; Holtz et al., 2015). CO2 can, therefore, passively diffuse through the vesicle membrane to replenish the carbonate ions consumed by calcification, thus sustaining calcification at moderate rates (see Figure 3 and Table 4 in Holtz et al., 2013) without additional metabolic costs. Additional carbon transport into the vesicles may only be required to sustain higher calcification rates, as observed in some coccolithophore species (e.g., G. huxleyi) that appeared later in the geological records. Data in support of these arguments can be found in Paasche (1962) and in Young and Ziveri (2000). Since the bicarbonate concentration in the calcifying vesicle is higher than in the cytoplasm, bicarbonate would then need to be transported actively under consumption of metabolic energy (Holtz et al., 2015). However, the bicarbonate gradient across the vesicle membrane is much smaller than the calcium gradient and CO2 can freely diffuse across the membrane, thereby functioning as a major source of carbon to the calcifying vesicle (Holtz et al., 2015) and, thus, preventing additional energetic requirements for bicarbonate transport.
3.3 Evolutionary success
Because of its low metabolic requirements, intracellular calcification does not require secondary evolutionary benefits to emerge. Yet, a number of hypotheses in relation to potential benefits of intracellular calcification have been proposed to explain both emergence and success of coccolithophores. These hypotheses include the potential enhancement of photosynthesis via the removal of carbonate ions (Nielsen, 1966; Paasche, 2002), protection against photoinhibition, advantages in hydrodynamic control, and protection against viruses, bacteria, and grazing (Monteiro et al., 2016). However, enhanced calcification does not enhance photosynthesis (Herfort et al., 2004; Trimborn et al., 2007) and non-calcifying coccolithophore cells can grow even faster than calcifying cells (Rost and Riebesell, 2004). Non-calcifying strains appear also not affected by photo-inhibition at high irradiances (Nanninga and Tyrrell, 1996). Additionally, coccolithophores use non-calcifying haploid stages to escape viral infections of calcified diploid cells (Frada et al., 2008), also questioning the relevance of calcification for viral protection. All these cases cast doubts on the assumption that calcification presents relevant physiological advantages.
Previous experiments (Müller et al., 2015) showed that calcifying cells can grow faster than non-calcifying cells when exposed to elevated calcium concentrations, indicating that intracellular calcification may protect against calcium poisoning (Simkiss, 1977). Our calculations show that the Ca2+-ATPase can transport ions against a gradient of up to 105 (see Supplementary Material S1). In non-calcifying cells, an increase of extracellular Ca2+ concentrations yields an increase of intracellular Ca2+ (Figure 2C). Since intracellular phosphate concentrations are usually around 4–5 mM (Auesukaree et al., 2004), cells operate at calcium and phosphate concentrations just below the solubility product of Ca3(PO4)2 (Chow, 2001). Increasing intracellular calcium concentrations, therefore, lead to precipitation with phosphate due to the low solubility of Ca3(PO4)2 (Pytkowicz and Kester, 1967; Chow, 2001). The decrease of cellular phosphate impedes cell growth in non-calcifying cells (Figure 2C). In calcifying cells, calcium transport into intracellular vesicles can still occur under elevated extracellular calcium concentrations because the calcium concentration inside the vesicle (ranging from 0.5 to 10 mM Holtz et al., 2013; Holtz et al., 2015) required for the precipitation of calcium carbonate is lower than outside the cell. The calcium gradient at the vesicle membrane therefore permits Ca2+-ATPase activity and allows maintenance of calcium homeostasis even under elevated extracellular calcium concentrations (Figure 2D). Cell division of calcifying cells remains unaffected (Müller et al., 2015).
Another benefit proposed for intracellular calcification is protection against zooplankton grazing (Jaya et al., 2016; Monteiro et al., 2016). However, coccolithophores are grazed by copepods without any significant sign of saturation of ingestion rates at increasing coccolithophore densities (Harris, 1994). This indicates that calcification and thus mechanical stability does not necessarily protect against copepod grazing. Calcification can, however, have an indirect negative effect on zooplankton grazing. Culture experiments (Harvey et al., 2015) showed that ingestion of calcifying cells reduces the growth rate of the grazers (Figure 2F), whereas ingestion of non-calcifying cells leads to an incrase in zooplankton growth rate (Figure 2E). A reduction of coccolithophore grazing mortality would thus provide calcifying cells with a competitive advantage against non-calcifying cells (Monteiro et al., 2016). Though not relevant for the emergence of pelagic nannoplankton calcification, the potential chemical protection against grazing may have contributed to its ecological success.
4 Conclusion
In summary, our analyses indicate that the first appearance of intracellular calcification is intrinsically linked to cellular calcium metabolism and to the prevention of calcium accumulation in the cytoplasm. This process did not require major physiological mutations or extra energy but only a very minor change, that is the activation of calcium transport proteins during their passage from the endoplasmic reticulum to the plasma membrane. Therefore, this innovation could have occurred at any time in the geologic past because preconditions were favourable, in line with evidence suggesting unrelated appearances in different lineages. A combination of environmental factors, such as chemical conditions and ecological interactions, may have subsequently helped calcifying nannoplankton to become abundant at a global scale. Therefore, nannoplankton calcifiers rose in pelagic environments during a time, the beginning of the Mesozoic, of profound changes in the structure of Earth’s ecosystems, marked by major climate changes and biological crises (i.e., the Permian–Triassic mass extinction, the Carnian Pluvial Episode and the Triassic-Jurassic mass extinction), which wiped out most of the Palaeozoic life and paved the way to the emergence of modern organisms. Within these new ecosystems, calcifying nannoplankton spread.
Data availability statement
The original contributions presented in the study are included in the article/Supplementary Material, further inquiries can be directed to the corresponding author.
Author contributions
SH, JDC, and AM conceived the idea of the study. SH and ST performed the energy budget calculations. KLH compiled the genetic information and performed the gene tree analysis. All authors contributed to the interpretation of the results. SH and AM wrote the manuscript with contributions from all authors. All authors contributed to the article and approved the submitted version.
Funding
Hanse-Wissenschaftskolleg (HWK) provided visiting fellowships to JDC and KLH.
Acknowledgments
We gratefully acknowledge the Hanse-Wissenschaftskolleg (HWK), Delmenhorst, Germany, for providing visiting fellowship to JDC and KLH and thus for creating the opportunity to bring together the multidisciplinary expertise necessary for this study.
Conflict of interest
The authors declare that the research was conducted in the absence of any commercial or financial relationships that could be construed as a potential conflict of interest.
Publisher’s note
All claims expressed in this article are solely those of the authors and do not necessarily represent those of their affiliated organizations, or those of the publisher, the editors and the reviewers. Any product that may be evaluated in this article, or claim that may be made by its manufacturer, is not guaranteed or endorsed by the publisher.
Supplementary material
The Supplementary Material for this article can be found online at: https://www.frontiersin.org/articles/10.3389/feart.2023.747059/full#supplementary-material
References
Auesukaree, C., Homma, T., Tochio, H., Shirakawa, M., Kaneko, Y., and Harashima, S. (2004). Intracellular phosphate serves as a signal for the regulation of the PHO pathway in Saccharomyces cerevisiae. J. Biol. Chem. 279, 17289–17294. doi:10.1074/jbc.M312202200
Benner, I., Diner, R. E., Lefebvre, S. C., Li, D., Komada, T., Carpenter, E. J., et al. (2013). Emiliania huxleyi increases calcification but not expression of calcification-related genes in long-term exposure to elevated temperature and pCO2. Philosophical Trans. R. Soc. B 368, 20130049. doi:10.1098/rstb.2013.0049
Berner, R. A., and Kothavala, Z. (2001). Geocarb III: A revised model of atmospheric CO2 over phanerozoic time. Am. J. Sci. 301, 182–204. doi:10.2475/ajs.301.2.182
Bown, P. R., Lees, J. A., and Young, J. R. (2004). “Calcerous nannoplankton evolution and diersity through time,” in Coccolithophores. From molecular processes to global impact (Springer Berlin), 481–508.
Boynton, A. L., Whitfield, F. F., and MacManus, J. P. (1980). Calmodulin stimulates DNA synthesis by Rat Liver cells. Biochem. Biophysical Res. Commun. 95, 745–749. doi:10.1016/0006-291x(80)90849-9
Bralower, T. J., Bown, P. R., and Siesser, W. G. (1991). Significance of upper triassic nannofossils from the southern hemisphere (ODP leg 122, wombat plateau, NW Australia). Mar. Micropaleontol. 17, 119–154. doi:10.1016/0377-8398(91)90025-2
Brownlee, C., Davies, M., Nimer, N., Dong, L., and Merrett, M. (1995). Calcification, photosynthesis and intracellular regulation in Emiliania huxleyi. Bull. l’institute Océanogr. Monaco 14, 19–36.
Brownlee, C., Wheeler, G. L., and Taylor, A. R. (2015). Coccolithophore biomineralization: New questions, new answers. Seminars Cell and Dev. Biol. 46, 11–16. doi:10.1016/j.semcdb.2015.10.027
Carafoli, E. (1987). Intracellular calcium homeostasis. Ann. Rev. Biochem. 56, 395–433. doi:10.1146/annurev.bi.56.070187.002143
Chow, L. (2001). Solubility of calcium phosphates. Octacalcium Phosphate l, 94–111. doi:10.1159/000061650
Dal Corso, J., Preto, N., Agnini, C., Hohn, S., Merico, A., Willems, H., et al. (2021). Rise of calcispheres during the carnian pluvial Episode (late triassic). Glob. Planet. Change 200, 103453. doi:10.1016/j.gloplacha.2021.103453
de Vargas, C., Aubry, M. P., Probert, I., and Young, J. (2007). Origin and evolution of coccolithophores. From coastal hunters to oceanic farmers. Evol. Prim. Prod. Sea 2007, 251–285. doi:10.1016/B978-012370518-1/50013-8
Demangel, I., Kovács, Z., Richoz, S., Gardin, S., Krystyn, L., Baldermann, A., et al. (2020). Development of early calcareous nannoplankton in the late triassic (northern calcareous alps, Austria). Glob. Planet. Change 193, 103254. doi:10.1016/j.gloplacha.2020.103254
Frada, M., Probert, I., Allen, M. J., Wilson, W. H., and de Vargas, C. (2008). The Cheshire Cat escape strategy of the coccolithophore Emiliania huxleyi in response to viral infection. Proc. Natl. Acad. Sci. 105, 15944–15949. doi:10.1073/pnas.0807707105
Gardin, S., Krystyn, L., Richoz, S., Bartolini, A., and Galbrun, B. (2012). Where and when the earliest coccolithophores? Lethaia 45, 507–523. doi:10.1111/j.1502-3931.2012.00311.x
Goddéris, Y., Donnadieu, Y., Vargas, C. D., Pierrehumbert, R. T., Dromart, G., and van de Schootbrugge, B. (2008). Causal or casual link between the rise of nannoplankton calcification and a tectonically-driven massive decrease in Late Triassic atmospheric CO2? Earth Planet. Sci. Lett. 267, 247–255. doi:10.1016/j.epsl.2007.11.051
Gussone, N., Langer, G., Thoms, S., Nehrke, G., Eisenhauer, A., Riebesell, U., et al. (2006). Cellular calcium pathways and isotope fractionation in Emiliania huxleyi. Geology 34, 625–628. doi:10.1130/G22733.1
Harris, R. P. (1994). Zooplankton grazing on the coccolithophore Emiliania huxleyi and its role in inorganic carbon flux. Mar. Biol. 119, 431–439. doi:10.1007/BF00347540
Harvey, E. L., Bidle, K. D., and Johnson, M. D. (2015). Consequences of strain variability and calcification in Emiliania huxleyi on microzooplankton grazing. J. Plankton Res. 37, fbv081–1148. doi:10.1093/plankt/fbv081
Hepler, P. K. (1994). The role of calcium in cell division. Cell Calcium 16, 322–330. doi:10.1016/0143-4160(94)90096-5
Herfort, L., Loste, E., Meldrum, F., and Thake, B. (2004). Structural and physiological effects of calcium and magnesium in Emiliania huxleyi (Lohmann) Hay and Mohler. J. Struct. Biol. 148, 307–314. doi:10.1016/j.jsb.2004.07.005
Holtz, L. M., Thoms, S., Langer, G., and Wolf-Gladrow, D. A. (2013). Substrate supply for calcite precipitation in Emiliania huxleyi: Assessment of different model approaches. J. Phycol. 49, 417–426. doi:10.1111/jpy.12052
Holtz, L. M., Wolf-Gladrow, D., and Thoms, S. (2015). Numerical cell model investigating cellular carbon fluxes in Emiliania huxleyi. J. Theor. Biol. 364, 305–315. doi:10.1016/j.jtbi.2014.08.040
Inesi, G., and Tadini-Buoninsegni, F. (2014). Ca2+/H+ exchange, lumenal Ca2+ release and Ca2+/ATP coupling ratios in the sarcoplasmic reticulum ATPase. J. Cell Commun. Signal. 8, 5–11. doi:10.1007/s12079-013-0213-7
Inouye, I., and Pienaar, R. N. (1983). Observations on the life cycle and microanatomy of Thoracosphaera heimii (Dinophyceae) with special reference to its systematic position. S. Afr. Tydskr. Plantk. 2, 63–75. doi:10.1016/S0022-4618(16)30147-4
Janofske, D. (1992). Kalkiges Nannoplankton, insbesondere Kalkige Dinoflagellaten-Zysten der alpinen Ober-Trias: Taxonomie, Biostratigraphie und Bedeutung für die Phylogenie der Peridiniales. Berl. Geowiss. Abh. Reihe E 4, 53.
Jantschke, A., Pinkas, I., Schertel, A., Addadi, L., and Weiner, S. (2020). Biomineralization pathways in calcifying dinoflagellates: Uptake, storage in mgcap-rich bodies and formation of the shell. Acta Biomater. 102, 427–439. doi:10.1016/j.actbio.2019.11.042
Jaya, B. N., Hoffmann, R., Kirchlechner, C., Dehm, G., Scheu, C., and Langer, G. (2016). Coccospheres confer mechanical protection: New evidence for an old hypothesis. Acta Biomater. 42, 258–264. doi:10.1016/j.actbio.2016.07.036
Kaźmierczak, J., Ittekkot, V., and Degens, E. T. (1985). Biocalcification through time: Environmental challenge and cellular response. Paläontologische Z. 59, 15–33. doi:10.1007/BF02985996
Kaźmierczak, J., and Kempe, (2004). “Calcium build-up in the precambrian sea,” in Origins: Genesis, evolution and diversity of life (Springer), 329–345.
Kaźmierczak, J., and Kremer, B. (2005). Early post-mortem calcified Devonian acritarchs as a source of calcispheric structures. Facies 51, 554–565. doi:10.1007/s10347-005-0071-8
Kearse, M., Moir, R., Wilson, A., Stones-havas, S., Cheung, M., Sturrock, S., et al. (2012). Geneious Basic: An integrated and extendable desktop software platform for the organization and analysis of sequence data. Bioinformatics 28, 1647–1649. doi:10.1093/bioinformatics/bts199
Knoll, A. H. (2003). Biomineralization and evolutionary history. Rev. Mineralogy Geochem. 54, 329–356. doi:10.2113/0540329
Krishtal, O. A., Pidoplichko, V. I., and Shakhovalov, Y. A. (1981). Conductance of the calcium channel in the membrane of snail neurones. J. physiology 310, 423–434. doi:10.1113/jphysiol.1981.sp013558
Langer, G., De Nooijer, L. J., and Oetjen, K. (2010). On the role of the cytoskeleton in coccolith morphogenesis: The effect of cytoskeleton inhibitors. J. Phycol. 46, 1252–1256. doi:10.1111/j.1529-8817.2010.00916.x
Langer, G., Gussone, N., Nehrke, G., Riebesell, U., Eisenhauer, A., Kuhnert, H., et al. (2006). Coccolith strontium to calcium ratios in Emiliania huxleyi: The dependence on seawater strontium and calcium concentrations. Limnol. Oceanogr. 51, 310–320. doi:10.4319/lo.2006.51.1.0310
Lewis, E., and Wallace, D. (1998). CO2SYS program. Tech. Rep., carbon dioxide information analysis center. Oak Ridge, Tennessee: Oak Ridge National Laboratory Environmental Sciences Division.
Lowenstam, H. A., and Margulis, L. (1980). Evolutionary prerequisites for early phanerozoic calcareous skeletons. BioSystems 12, 27–41. doi:10.1016/0303-2647(80)90036-2
Lux, H. D., and Nagy, K. (1981). Single Channel Ca2+ currents in helix pomatia neurons. Pflügers Archiv Eur. J. Physiology 391, 252–254. doi:10.1007/bf00596179
Marcum, J. M., Dedman, J. R., Brinkley, B. R., and Means, A. R. (1978). Control of microtubule assembly-disassembly by calcium-dependent regulator protein. Proc. Natl. Acad. Sci. 75, 3771–3775. doi:10.1073/pnas.75.8.3771
Meier, K. J., Young, J. R., Kirsch, M., and Feist-Burkhardt, S. (2007). Evolution of different life-cycle strategies in oceanic calcareous dinoflagellates. Eur. J. Phycol. 42, 81–89. doi:10.1080/09670260600937833
Meldolesi, J., and Pozzan, T. (1998). The endoplasmic reticulum Ca2+ store: A view from the lumen. Trends Biochem. Sci. 23, 10–14. doi:10.1016/S0968-0004(97)01143-2
Montañez, I. P., McElwain, J. C., Poulsen, C. J., White, J. D., DiMichele, W. A., Wilson, J. P., et al. (2016). Climate, pco2 and terrestrial carbon cycle linkages during late palaeozoic glacial–interglacial cycles. Nat. Geosci. 9, 824–828. doi:10.1038/ngeo2822
Monteiro, F. M., Bach, L. T., Brownlee, C., Bown, P., Rickaby, R. E. M., Poulton, A. J., et al. (2016). Why marine phytoplankton calcify. Sci. Adv. 2, e1501822. doi:10.1126/sciadv.1501822
Müller, M. N., Barcelos E Ramos, J., Schulz, K. G., Riebesell, U., Kaźmierczak, J., Gallo, F., et al. (2015). Phytoplankton calcification as an effective mechanism to alleviate cellular calcium poisoning. Biogeosciences 12, 6493–6501. doi:10.5194/bg-12-6493-2015
Nanninga, H. J., and Tyrrell, T. (1996). Importance of light for the formation of algal blooms by Emiliania huxleyi. Mar. Ecol. Prog. Ser. 136, 195–203. doi:10.3354/meps136195
Nielsen, E. S. (1966). The uptake of free CO2 and HCO3- during photosynthesis of plankton algae with special reference to the coccolithophorid coccolithus huxleyi. Physiol. Plant. 19, 232–240. doi:10.1111/j.1399-3054.1966.tb09095.x
Onoue, T., and Yoshida, A. (2010). Depositional response to the Late Triassic ascent of calcareous plankton in pelagic mid-oceanic plate deposits of Japan. J. Asian Earth Sci. 37, 312–321. doi:10.1016/j.jseaes.2009.08.013
Orrenius, S., McConkey, D. J., Bellomo, G., and Nicotera, P. (1989). Role of Ca2+ in toxic cell killing. Trends Pharmacol. Sci. 10, 281–285. doi:10.1016/0165-6147(89)90029-1
Paasche, E. (2002). A review of the coccolithophorid Emiliania huxleyi (Prymnesiophyceae), with particular reference to growth, coccolith formation, and calcification-photosynthesis interactions. Phycologia 40, 503–529. doi:10.2216/i0031-8884-40-6-503.1
Pfeffer, S. R., and Rothmann, J. E. (1987). Biosynthetic protein transport and sorting by the endoplasmic reticulum and golgi. Annu. Rev. Biochem. 56, 829–852. doi:10.1146/annurev.bi.56.070187.004145
Poenie, M., Alderton, J., Tsien, R. Y., and Steinhardt, R. A. (1985). Changes of free calcium levels with stages of the cell division cycle. Nature 315, 147–149. doi:10.1038/315147a0
Preto, N., Agnini, C., Rigo, M., Sprovieri, M., and Westphal, H. (2013a). The calcareous nannofossil Prinsiosphaera achieved rock-forming abundances in the latest Triassic of Western Tethys: Consequences for the δ13C of bulk carbonate. Biogeosciences 10, 6053–6068. doi:10.5194/bg-10-6053-2013
Preto, N., Willems, H., Guaiumi, C., and Westphal, H. (2013b). Onset of significant pelagic carbonate accumulation after the carnian pluvial event (CPE) in the Western tethys. Facies 59, 891–914. doi:10.1007/s10347-012-0338-9
Pytkowicz, R. M., and Kester, D. R. (1967). Relative calcium phosphate saturation in two regions of the north pacific ocean. Limnol. Oceanogr. 12, 714–718. doi:10.4319/lo.1967.12.4.0714
Raup, D., and Sepkoski, J. (1982). Mass extinctions in the marine fossil record. Science 215, 1501–1503. doi:10.1126/science.215.4539.1501
Read, B. A., Kegel, J., Klute, M. J., Kuo, A., Lefebvre, S. C., Maumus, F., et al. (2013). Pan genome of the phytoplankton Emiliania underpins its global distribution. Nature 499, 209–213. doi:10.1038/nature12221
Ridgwell, A. (2005). A Mid Mesozoic Revolution in the regulation of ocean chemistry. Mar. Geol. 217, 339–357. doi:10.1016/j.margeo.2004.10.036
Ridgwell, A., and Zeebe, R. E. (2005). The role of the global carbonate cycle in the regulation and evolution of the Earth system. Earth Planet. Sci. Lett. 234, 299–315. doi:10.1016/j.epsl.2005.03.006
Rost, B., and Riebesell, U. (2004). “Coccolithophores and the biological pump: Responses to environmental changes,” in Coccolithophores: From molecular processes to global impact. Editors H. R. Thierstein, and J. R. Young (Springer Berlin), 99–125. doi:10.1007/978-3-662-06278-4_5
Royer, D. L., Berner, R. A., Montañez, I. P., Tabor, N. J., and Beerling, D. J. (2004). CO2 as a primary driver of Phanerozoic climate. Geol. Soc. Am. Today 14, 4–10. doi:10.1130/1052-5173(2004)014<4:caapdo>2.0.co;2
Sanders, D., Pelloux, J., Brownlee, C., and Harper, J. F. (2002). Calcium at the crossroads of signaling. Plant Cell 14 (l), S401–S417. doi:10.1105/tpc.002899
Sepkoski, J. J., and Ausich, W. I. (1990). “Evolutionary faunas,” in Palaeobiology: A synthesis. Editors D. E. G. Briggs, and P. R. Crowther (Oxford: Blackwell Scientific Publications), 37–41.
Simkiss, K. (1977). Biomineralization and detoxification. Calcif. Tissue Res. 24, 199–200. doi:10.1007/BF02223316
Stanley, S. M., and Hardie, L. A. (1998). Secular oscillations in the carbonate mineralogy of reef-building and sediment-producing organisms driven by tectonically forced shifts in seawater chemistry. Palaeogeogr. Palaeoclimatol. Palaeoecol. 144, 3–19. doi:10.1016/S0031-0182(98)00109-6
Steinhardt, R. A., and Alderton, J. (1988). Intracellular free calcium rise triggers nuclear envelope breakdown in the sea urchin embryo. Nature 332, 364–366. doi:10.1038/332364a0
Strom, S., Wolfe, G., Holmes, J., Stecher, H., Shimeneck, C., and Sarah, L. (2003). Chemical defense in the microplankton i: Feeding and growth rates of heterotrophic protists on the dms-producing phytoplankter emiliania huxleyi. Limnol. Oceanogr. 48, 217–229. doi:10.4319/lo.2003.48.1.0217
Tidow, H., Poulsen, L. R., Andreeva, A., Knudsen, M., Hein, K. L., Wiuf, C., et al. (2012). A bimodular mechanism of calcium control in eukaryotes. Nature 491, 468–472. doi:10.1038/nature11539
Toyoshima, C., and Inesi, G. (2004). Structural basis of ion pumping by Ca2+-ATPase of the sarcoplasmic reticulum. Annu. Rev. Biochem. 73, 269–292. doi:10.1146/annurev.biochem.73.011303.073700
Trimborn, S., Langer, G., and Rost, B. (2007). Effect of varying calcium concentrations and light intensities on calcification and photosynthesis in Emiliania huxleyi. Limnol. Oceanogr. 52, 2285–2293. doi:10.4319/lo.2007.52.5.2285
Tsien, R. W. (1983). Calcium channels in excitable cell membranes. Annu. Rev. Physiology 45, 341–358. doi:10.1146/annurev.ph.45.030183.002013
Verret, F., Wheeler, G., Taylor, A. R., Farnham, G., and Brownlee, C. (2010). Calcium channels in photosynthetic eukaryotes: Implications for evolution of calcium based signalling. New Phytol. 187, 23–43. doi:10.1111/j.1469-8137.2010.03271.x
von Dassow, P., Ogata, H., Probert, I., Wincker, P., Da Silva, C., Audic, S., et al. (2009). Transcriptome analysis of functional differentiation between haploid and diploid cells of Emiliania huxleyi, a globally significant photosynthetic calcifying cell. Genome Biol. 10, R114. doi:10.1186/gb-2009-10-10-r114
Winther, A.-M. L., Bublitz, M., Karlsen, J. L., Møller, J. V., Hansen, J. B., Nissen, P., et al. (2013). The sarcolipin-bound calcium pump stabilizes calcium sites exposed to the cytoplasm. Nature 495, 265–269. doi:10.1038/nature11900
Witkowski, C. R., Weijers, J. W., Blais, B., Schouten, S., and Sinninghe Damsté, J. S. (2018). Molecular fossils from phytoplankton reveal secular Pco2 trend over the Phanerozoic. Sci. Adv. 4, eaat4556. doi:10.1126/sciadv.aat4556
Young, J. R. (2003). Biomineralization within vesicles: The calcite of coccoliths. Rev. Mineralogy Geochem. 54, 189–215. doi:10.2113/0540189
Young, J. R., Geisen, M. P. I., and Probert, I. (2005). A review of selected aspects of coccolithophore biology with implications for paleobiodiversity estimation. Micropaleontology 51, 267–288. doi:10.2113/gsmicropal.51.4.267
Young, J. R., and Ziveri, P. (2000). Calculation of coccolith volume and it use in calibration of carbonate flux estimates. Deep-Sea Res. Part II Top. Stud. Oceanogr. 47, 1679–1700. doi:10.1016/S0967-0645(00)00003-5
Keywords: calcareous nannoplankton, unicellular eukaryotes, calcium homeostasis, ion transport, Carnian, coccolithophores
Citation: Hohn S, Dal Corso J, Hoke KL, Thoms S and Merico A (2023) Environmental and physiological conditions that led to the rise of calcifying nannoplankton in the Late Triassic. Front. Earth Sci. 11:747059. doi: 10.3389/feart.2023.747059
Received: 25 July 2021; Accepted: 17 July 2023;
Published: 31 July 2023.
Edited by:
Ángel Puga-Bernabéu, University of Granada, SpainReviewed by:
Annette Goetz, State Authority for Mining, Energy and Geology, GermanyWolfram Michael Kürschner, University of Oslo, Norway
Copyright © 2023 Hohn, Dal Corso, Hoke, Thoms and Merico. This is an open-access article distributed under the terms of the Creative Commons Attribution License (CC BY). The use, distribution or reproduction in other forums is permitted, provided the original author(s) and the copyright owner(s) are credited and that the original publication in this journal is cited, in accordance with accepted academic practice. No use, distribution or reproduction is permitted which does not comply with these terms.
*Correspondence: Agostino Merico, agostino.merico@leibniz-zmt.de