Abstract
Survival of larval Antarctic krill (Euphausia superba) during winter is largely dependent upon the presence of sea ice as it provides an important source of food and shelter. We hypothesized that sea ice provides additional benefits because it hosts fewer competitors and provides reduced predation risk for krill larvae than the water column. To test our hypothesis, zooplankton were sampled in the Weddell-Scotia Confluence Zone at the ice-water interface (0–2 m) and in the water column (0–500 m) during August–October 2013. Grazing by mesozooplankton, expressed as a percentage of the phytoplankton standing stock, was higher in the water column (1.97 ± 1.84%) than at the ice-water interface (0.08 ± 0.09%), due to a high abundance of pelagic copepods. Predation risk by carnivorous macrozooplankton, expressed as a percentage of the mesozooplankton standing stock, was significantly lower at the ice-water interface (0.83 ± 0.57%; main predators amphipods, siphonophores and ctenophores) than in the water column (4.72 ± 5.85%; main predators chaetognaths and medusae). These results emphasize the important role of sea ice as a suitable winter habitat for larval krill with fewer competitors and lower predation risk. These benefits should be taken into account when considering the response of Antarctic krill to projected declines in sea ice. Whether reduced sea-ice algal production may be compensated for by increased water column production remains unclear, but the shelter provided by sea ice would be significantly reduced or disappear, thus increasing the predation risk on krill larvae.
Similar content being viewed by others
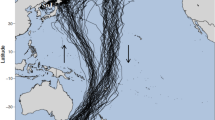
Avoid common mistakes on your manuscript.
Introduction
Antarctic krill (Euphausia superba, Dana 1850, hereafter ‘krill’) is a key species in the Southern Ocean, supporting large populations of top predators and an important commercial fishery (McBride et al. 2014; Krafft et al. 2015). Recruitment success of krill depends on larval survival during their first winter (Atkinson et al. 2004, 2019; Meyer et al. 2009). Current knowledge of larval krill ecology in winter, however, is based on a limited number of winter studies (Marschall 1988; Daly 2004; Ross et al. 2004; Meyer et al. 2017; Schaafsma et al. 2017). Unlike Antarctic krill adults, krill larvae have low lipid reserves (Hagen and Auel 2001; Kohlbach et al. 2017) and can survive only a few weeks without food (Ross and Quetin 1991; Meyer et al. 2002). The ice-water interface is considered to be a key overwintering habitat for krill (Meyer et al. 2009; Flores et al. 2012), providing shelter and ice algae as an important food source (Daly and Macaulay 1991; Quetin and Ross 1991; Meyer et al. 2017; Schaafsma et al. 2017). Recent studies have demonstrated that despite a diverse diet, the overwhelming proportion of carbon in overwintering krill larvae originates from ice algae production (Kohlbach et al. 2017), and that ice algae-derived carbon is also important in the carbon budget of various zooplankton species (Kohlbach et al. 2018). Besides ice algae, other sea ice-derived resources such as protozoans, small copepods and detritus may offer an alternative food source during winter for krill larvae and other pelagic species dwelling at the ice-water interface (Daly 1990; Meyer 2012; Schmidt et al. 2014; Schaafsma et al. 2017).
Some Antarctic copepods actively feed in the upper epipelagic layer during winter, including Calanus propinquus, Metridia gerlachei and Stephos longipes (Bathmann et al. 1993; Drits et al. 1993; Pasternak and Schnack-Schiel 2001, 2007). Therefore, these copepods are potentially competing for food resources with the Antarctic krill larvae. Copepods are regarded as dominant grazers (Atkinson et al. 1996; Bernard and Froneman 2003; Pakhomov and Froneman 2004) and, in some regions, they can control the phytoplankton standing stocks (Granĺi et al. 1993; Froneman et al. 2000b; Urban-Rich et al. 2001). In the Southern Ocean, copepod grazing studies are mostly limited to observations during spring, summer or autumn, when primary production in the water column is high (Atkinson et al. 1996; Swadling et al. 1997; Pakhomov and Froneman 2004; Bernard et al. 2012; Lee et al. 2013). Feeding in winter is expected to be lower, because less autotrophic food is available (Bathmann et al. 1993; Hopkins et al. 1993). Alternative winter strategies were suggested for the actively feeding copepods, such as omnivorous feeding and partially compensating with lipid storage (Torres et al. 1994; Metz and Schnack-Schiel 1995; Hagen and Auel 2001).
Alternative feeding strategies in winter are not as essential for carnivores because of the presence of zooplankton year-round, however, predators might actively search and follow the prey, which may influence their vertical distribution (Torres et al. 1994, Quetin et al. 1996). For example, feeding below the epipelagic layer during winter seems advantageous for gammarid amphipods (Torres et al. 1994), as the bulk of zooplankton is found in the deeper water column (Schnack-Schiel and Hagen 1995). Chaetognaths are also known to feed year-round on copepods and euphausiid larvae (Lancraft et al. 1991; Froneman et al. 1998; Kruse et al. 2010b; Giesecke and González 2012). Their feeding intensity during winter is no less than summer estimates (Oresland 1995), being capable of consuming up to 5% of the mesozooplankton standing stocks (Oresland 1995; Froneman and Pakhomov 1998). Gelatinous zooplankton can also insert large predation impact on mesozooplankton (Purcell 1991; Gibbons et al. 1992; Mills 1995) and can reach significant levels under the ice in winter (Flores et al. 2011). One abundant ctenophore of the Southern Ocean, Callianira antarctica, was shown to selectively feed on krill larvae and copepods (Scolardi et al. 2006). In the Scotia-Weddell Confluence Zone, divers reported ctenophores preying under ice on the overwintering larval Antarctic krill (Daly and Macaulay 1991).
In this study we hypothesize that fewer competitors and predators at the ice-water interface are key benefits provided by the sea ice habitat for larval krill compared to the underlying water column. To test our hypotheses, we compared (1) the abundance of dominant competitors and their grazing impact; and (2) the predation risk by dominant carnivorous macrozooplankton between the two habitats. This study was based on the RV Polarstern expedition PS81, which was conducted in the winter/early spring of 2013. The abundance and biomass distribution of larval krill between the two habitats, the ice-water interface and the water column, was described in Schaafsma et al. (2016). The zooplankton data collected at the ice-water interface were presented in David et al. (2017). In this study we complete the picture by adding the zooplankton data collected in the water column during the same expedition. In addition to comparing larval krill distribution between the two habitats, here we also describe and compare the presence and abundance of their competitors and predators. By doing this, we aim to highlight the benefits provided by the sea ice as a winter habitat for larval krill.
Material and methods
Sampling technique and data collection
Sampling was performed during RV Polarstern expedition PS81 (ANT XXIX/7), between 31 August and 2 October 2013, under the pack-ice in the Weddell Sea, from 58° to 61°S, and from 42° to 25°W (Fig. 1). At 11 stations, the ice-water interface layer (0–2 m) was sampled with a Surface and Under-Ice Trawl (SUIT; van Franeker et al. 2009). Seven SUIT stations were carried out from west to east approximately along the 60°S parallel, and four stations northward along the 26°W meridian (Table 1; Fig. 1).
Stations map during RV Polarstern expedition PS81 ANT XXIX/7. Sampling was performed from west to east, from August to October 2013. Number codes next to sampling locations indicate sampling gear [S Surface and Under-Ice Trawl (SUIT), R Rectangular Midwater Trawl (RMT) and C Conductivity Temperature Depth probe (CTD)] and station numbers. Sea-ice concentration showed in the background was acquired from Bremen University (http://www.iup.uni-bremen.de: 8084/amsr/) with white areas indicative of open water. The monthly mean sea-ice extent for September 2013 was acquired from NSIDC (Fetterer et al. 2017)
The SUIT consists of a steel frame with a 2 m × 2 m opening and two parallel 15 m long nets attached, including: (1) a 7-mm half-mesh commercial shrimp net, lined with 0.3-mm mesh in the rear 3 m of the net, covering 1.5 m of the opening width; and (2) a 0.3-mm mesh mesozooplankton net covering 0.5 m of the opening width. Floats attached to the top of the frame keep the net at the sea-ice underside. An asymmetric bridle forces the net to tow off at an angle of approximately 60° to starboard of the ship’s track, at a cable length of 150 m, to ensure sampling outside of the ship’s wake. A detailed description of the SUIT sampling technique and performance was provided in Flores et al. (2012). Depending on the sea ice conditions, SUIT haul durations varied between 17 and 42 min (mean = 29 min) over an average distance of 1.5 km (range 0.5–3 km). Water inflow speed was estimated using a Nortek Aquadopp® Acoustic Doppler Current Profiler (ADCP). The trawled area was calculated by multiplying the distance sampled in water, calculated from ADCP data, with the net width (0.5 m for the zooplankton net, and 1.5 m for the shrimp net). A detailed description of SUIT operations during PS81 was provided in David et al. (2017).
Nine Rectangular Midwater Trawl (RMT) stations were conducted in close proximity to the horizontal SUIT hauls (Table 1). The 0–500 m layer was sampled with double oblique hauls using the RMT. The trawl consisted of two nets: (1) an RMT-8 with a mesh size of 4.5 mm at the opening and 0.85 mm at the cod-end with a mouth opening of 8 m2; and (2) an RMT-1 with a 0.33-mm mesh and an opening of 1 m2. A mechanical impeller flowmeter (Hydro Bios, Kiel) was mounted in the mouth of the RMT-8 to measure the volume of filtered water. The volume of water filtered by the RMT-1 ranged between 1055 and 4280 m3, and between 10,547 and 53,236 m3 by the RMT-8. Further details of RMT sampling are given in Schaafsma et al. (2016).
Environmental data
A Conductivity Temperature Depth probe (CTD; Sea and Sun CTD75M) with built-in fluorometer was mounted in the SUIT frame to collect environmental parameters from the ice-water interface (0–2 m). An altimeter (Tritech PA500/6-E) was connected to the CTD probe and measured the distance between the net and the sea-ice underside, from which sea-ice thickness was derived. Two radiometers (RAMSES, TriOS) were mounted on the SUIT to record hyperspectral profiles of incoming light during the hauls. At three SUIT stations there was sufficient daylight to estimate the chlorophyll a concentration (chl a) of sea-ice algae from hyperspectral light profiles. Details on environmental-data collection and processing from the ice-water interface are found in Lange et al. (2016) and Castellani et al. (2020).
Gridded daily sea-ice concentrations for the Southern Ocean derived from AMSR2 satellite data, using the algorithm specified by Spreen et al. (2008), were downloaded from the sea-ice portal hosted at University Bremen (www.meereisportal.de).
A CTD-probe (Seabird SBE911 +) attached to a carousel water sampler was used to collect environmental parameters from the water column (0–250 m). The CTD was equipped with a fluorometer (Wetlabs FLRTD) and a transmissometer (Wetlabs C-Star). Among all CTD stations, the closest in time and space to the SUIT and RMT stations were chosen (Table 1, Fig. 1). Chl a concentrations of water samples were measured with a Turner 10-AU fluorometer. Calibration of the fluorometer with a range of concentrations of chl a standard (from Anacystis nidulans, Sigma-Aldrich) was carried out at the beginning and at the end of the cruise. For further details of chl a measurements see Meyer et al. (2017).
Biological data
The zooplankton catch was partially sorted on board and some animals were immediately extracted and frozen at − 20 °C for further molecular analysis. A quantitative subsample of the material was preserved in 4% formaldehyde/seawater solution. After the expedition, the preserved samples from SUIT hauls were analyzed for species composition and abundance at the Alfred Wegener Institute, Germany. Samples from RMT hauls were analyzed at the University of British Columbia, Canada. Specimens of mesozooplankton and chaetognaths were used from the SUIT mesozooplankton net and from the RMT-1. For carnivores, other than chaetognaths, specimens from the SUIT shrimp net and from the RMT-8 were used. Large macrozooplankton were first extracted from the sample and all specimens were counted. The remainder of the sample was then divided into smaller fractions with a Motoda plankton splitter to facilitate counting of dominant species. Dominant species were counted in the smallest fraction in which they reach at least 100 individuals, then the count was multiplied with the fraction to reach the total number in the sample. The less abundant and rare species were counted in the entire sample. All copepods were identified and counted per species and development stage. Adult copepods and their copepodite stages were then integrated per species in abundance calculations. Areal abundances from horizontal hauls were calculated by dividing the total number of animals per haul by the trawled area. For RMT oblique hauls, volumetric abundances were calculated by dividing the total number of animals per haul by the volume of water filtered. Conversion to areal abundances was done by multiplying volumetric abundances by the sampling depth (Table 1).
In the case of macrozooplankton species, total body length was measured to the nearest 0.1 mm and a mean size per species was used for biomass calculations. Zooplankton biomass was calculated using species length–weight relationships and was expressed as mg dry weight m−2 (Mizdalski 1988). To estimate biomass for copepod species, a mean dry weight was theoretically assumed for each developmental stage and multiplied to abundances using the stage distribution of each species (Mizdalski 1988). Krill catches were staged according to Makorov and Denys (1981) and Kirkwood (1982). Details on staging, analysis of length–stage and biomass calculations are presented in Schaafsma et al. (2016).
Data analysis
Conversion of chl a to carbon units was performed applying a carbon:chl a equivalent ratio of 50 (Atkinson et al. 1996). The phytoplankton standing stock at the ice-water interface was estimated from chl a data measured with the CTD (Sea and Sun CTD75M) attached to the SUIT and integrated over 0–2 m depth. The phytoplankton standing stock in the water column was estimated from chl a data measured with the vertical CTD (Seabird SBE911 +) attached to the carousel water sampler and was calculated as depth-integrated chl a biomass from the surface to 250 m depth \(\left( {\sum\nolimits_{z = 1}^{250} {{\text{chl}}\;a_{z} } } \right)\). The chl a values in the water column decreased to nearly zero by 200 m depth, therefore the integrated values over the 0–250 m were considered largely representative for the entire water column.
Daily ingestion rates (mg C m−2 d−1) at the ice-water interface (0–2 m) and in the water column (0–500 m) were calculated for larval Antarctic krill and for abundant copepod species (C. propinquus, Ctenocalanus spp., M. gerlachei, Oithona similis and S. longipes) by multiplying reported individual ingestion rates (µg C ind−1 d−1; Table 2) with each species' areal abundance (ind. m−2; Table 3). With the exception of larval Antarctic krill and C. propinquus, we found a general lack of data on ingestion rates during wintertime. Therefore, for the species lacking such information, we inferred winter ingestion rates from summer-spring estimates based on the following assumptions: (1) ingestion rates in winter are a fraction of the summer rates, which is proportional to the decrease in metabolic rates with a decrease in temperature, (2) the same fraction is assumed for all actively-feeding co-occurring species, (3) all grazers were feeding primarily on phytoplankton, ignoring the presence of other potential resources. The ingestion rates of copepods are expected to decrease during winter as their metabolism slows down with lowering temperatures (Ikeda 1985). The ingestion values for the actively-feeding copepods were reduced by 50% in the present study to account for winter slow down. This reduction is assumed to be a conservative estimate to account for differences between basal standard and active metabolism in zooplankton species (Torres et al. 1982; Torres and Childress 1983). We justify our choice of reduction by 50% based on the example of C. propinquus, for which both summer and winter ingestion rates were reported. Drits et al. 1993 reported summer ingestion rates of about 10 µg C ind−1 d−1 (range 2.8–23.4) at high chl a concentration, while Bathmann et al. 1993 reported winter ingestion rates of 5 µg C ind−1 d−1 at low chl a concentration and 10–19 µg C ind−1 d−1 at high chl a concentration (estimated using the same C:chl a ratio of 50). Nevertheless, due to uncertainty in winter estimates for the other species in this study, we also performed a sensitivity analysis to test the effect of the reduction in feeding by 25%, 50% and 75% on the total community grazing. In addition, we tested the robustness of our total community grazing estimates to variations in each species ingestion rates separately with an Individual Parameter Perturbation analysis (Online resource 1).
Grazing impact per species was estimated at the ice-water interface and in the water column as a percentage of the phytoplankton standing stock grazed daily:
During winter, the bulk of the carbon uptake in C. propinquus, Antarctic krill larvae and other zooplankton is derived from ice algae (Kohlbach et al. 2017, 2018). Because ice-algae biomass could only be measured at three SUIT stations due to low light, we limited our assessment of grazing impact to phytoplankton standing stock, under the assumption that chl a concentration in the ice-water interface layer was also indicative of suspended ice algae released from sea ice.
We used individual ingestion rates of dominant carnivorous macrozooplankton multiplied with each species' areal abundance (ind. m−2) to estimate predation risk (Table 2). Predation risk by taxonomic group was expressed as a percentage of the mesozooplankton standing stock.
In the case of euphausiids, i.e., Thysanoessa macrura, and cnidarians the predation risk was calculated as a percentage of mesozooplankton biomass because the ingestion rates for these organisms were reported as % of individual body weight (Table 2). The mesozooplankton standing stock was estimated at the ice-water interface from the SUIT-mesozooplankton net and in the water column from the RMT1-net. For one Beroe sp. found in the SUIT samples and for one unidentified ctenophore found in the RMT samples we used the same ingestion rate as C. antarctica. Wintertime ingestion rates of siphonophores were unavailable, but because other cnidarians generally showed comparable feeding rates across seasons, we assume that these were acceptable to use as they are.
Statistical differences in grazing impact and predation risk between the ice-water interface and the water column habitats were assessed with the non-parametric Wilcoxon rank sum test (Wilcoxon 1945). Statistical analyses were performed with the software R, version 3.5.0 (R-Development-Core-Team 2016).
Results
Environmental conditions
All sampled stations were sea-ice covered. Satellite-derived sea-ice coverage at the sampling locations, averaged over a pixel size of 6.25 km × 6.25 km, was in general between 86 and 100%. Only at two stations (S570_5 and S579_2), sampled north of 60°S at the end of September/beginning of October, was sea-ice coverage about 50%, placing them in the vicinity of the Marginal Ice Zone (Table 1, Fig. 1). Further details of sea ice, water parameters and snow cover are given in David et al. (2017) and Castellani et al. (2020).
At the ice-water interface (0–2 m layer), chl a concentrations ranged from 0.10 to 0.13 mg chl a m−3 south of 60°S during August/early-September and showed higher values ranging from 0.16 to 0.27 mg chl a m−3 north of 60°S during late-September/beginning of October (Table 1; Fig. 2). In-ice chl a values at stations S555_47, S565_5 and S577_2 were about 8 mg chl a m−2 (Castellani et al. 2020). In the water column, the volumetric chl a concentration in the upper 100 m layer, indicative of the winter mixed layer depth (Pellichero et al. 2017) was within the same range as the ice-water interface values, ranging between 0.04 and 0.33 mg chl a m−3 (Fig. 2). The values in the deeper water column decreased sharply below 100 m, reaching nearly zero at 200 m depth, therefore the integrated values over 0–250 m were considered representative of the entire water column. Depth-integrated chl a concentrations in the water column (0–250 m depth) ranged between 8.46 and 35.45 mg chl a m−2 (Table 4).
Volumetric (a) and areal (b) chlorophyll a concentrations (Chl a) at the ice-water interface [measured with the SUIT (Surface and Under-Ice Trawl)—CTD (Conductivity Temperature Depth probe)] and in the water column (measured with the water column CTD cast). For measurements at the ice-water interface, a represents values averaged over the 0–2 m depth layer, while b represents values integrated over the 0–2 m depth layer. For measurements in the water column, a represents values averaged over the 0–100 m depth layer, and b represents values integrated over the 0–250 m depth layer
Zooplankton at the ice-water interface
At the ice-water interface (0–2 m layer), the copepod species C. propinquus, Ctenocalanus spp., M. gerlacheii, S. longipes and O. similis numerically accounted for 64% of the total zooplankton community. In all these species, the dominant stage was CV. The Antarctic krill larvae were the second most abundant group, comprising 25% of the total abundance (Table 3). Even though the numerical contribution of M. gerlachei and O. similis to the ice-water interface community was less than 1%, they were selected for being among the most abundant copepods in the water column. Krill larvae abundance in the 0–2 m layer varied between 0.01 and 3.57 ind. m−2, with a median abundance of 0.29 ind. m−2 (Table 3). The smaller species S. longipes and Ctenocalanus spp. had the highest copepod abundances at the ice-water interface of 1.04 ind. m−2 and 0.23 ind. m−2, respectively, followed by the larger species C. propinquus with 0.16 ind. m−2 (Table 3). In terms of biomass, the selected copepod species represented 17% of the total ice-water interface community, with higher contributions (up to 33%) at stations S560_2–S570_5. Krill larvae had higher biomass than copepods, on average 25% of the total mesozooplankton standing stock in the ice-water interface layer, while juveniles and sub-adult krill together represented an additional 35% of the biomass.
Dominant carnivorous zooplankton at the ice-water interface consisted of amphipods, chaetognaths, ctenophores and siphonophores. The amphipods and chaetognaths numerically accounted for only 0.57 and 0.33% of the ice-water interface community, yet each group represented about 4% of the total biomass. The most abundant amphipod was C. lucasii with 0.0020 ind. m−2, followed by Primno macropa with 0.0006 ind. m−2. The most abundant chaetognath was E. hamata (0.0060 ind. m−2), followed by Sagitta spp. (0.0042 ind. m−2), which accounted for half of E. hamata’s median abundance (Table 3). Ctenophores were present in low abundance at the ice-water interface at only two stations, accounting for 10% and 15% of the biomass, respectively. Siphonophores comprised 0.31 and 1.45% of the abundance and biomass of the ice-water interface community (Table 3). A detailed description of zooplankton species composition, abundance and biomass at the ice-water interface was presented by David et al. (2017).
Zooplankton in the water column
In the water column (0–500 m layer), the dominant copepod species contributed 60% (numerically) and 20% (by mass) to the mesozooplankton community, while the numerical contribution of krill larvae was negligible (< 1%; Table 3). The areal abundance of krill larvae in the top 500 m varied between 0 and 33 ind. m−2, with a median abundance of 2.5 ind. m−2. The small copepod species O. similis and Ctenocalanus spp. had the highest areal abundances in the water column at 2875 ind. m−2 and 1415 ind. m−2, respectively, followed by the larger species M. gerlachei (1039 ind. m−2; Table 3). The copepod Calanoides acutus was added to the mesozooplankton stock in the water column for predation risk estimates, but because this species enters diapause in winter (Atkinson 1991; Bathmann et al. 1993) it was not used in grazing estimates.
Carnivorous zooplankton in the water column, besides amphipods and chaetognaths, was largely comprised of gelatinous species. Chaetognaths numerically accounted for 7% of the zooplankton community, which was dominated by E. hamata, both in terms of abundance and biomass (649 ind. m−2; 1803 mg DW m−2), in comparison to Sagitta spp. (56 ind. m−2; 1120 mg DW m−2). Numerically, amphipods and ctenophores represented only a small fraction of the community at 0.47% and 0.16%, respectively. The biomass of amphipods was small compared to the ctenophores, which reached a maximum contribution of 15% of the total biomass at station R570_1. The dominant contributors to the carnivorous zooplankton community in the water column in terms of biomass were medusae and siphonophores at 50% and 30%, respectively (Table 3).
Grazing impact
At the ice-water interface (0–2 m) grazing by dominant mesozooplankton species, expressed as a percentage of phytoplankton standing stock consumed daily, varied between < 0.01% at station S577_2 and 0.28% at station S562_5 (Table 4). Copepod grazing was the highest at most stations, with a nearly equal grazing contribution from copepods and krill larvae at the three most north-eastern stations. The highest krill larvae grazing of 0.07% of the phytoplankton standing stock was encountered at the south-eastern stations S565_5 and S567_2. Similarly, at station S562_5 the highest copepod grazing was observed accounting for 0.28% of the phytoplankton standing stock.
In the water column (0–500 m), grazing impact varied between 0.51% of the phytoplankton standing stock at station R548_5 and 5.62% at station R565-12, and was largely dominated by copepods over the entire sampled area (Table 4). Grazing by krill larvae was within the same range at the ice-water interface and in the water column (Wilcoxon rank sum test, W = 71, p = 0.110). However, grazing of the entire community was significantly higher in the water column than at the ice-water interface, due to the high number of pelagic copepods (Wilcoxon rank sum test, W = 1, p < 0.001; Fig. 3). Assuming that all grazers would have been concentrated in the upper 250 m (i.e. half of the water-column habitat) where phytoplankton resources were available, would imply a reduction in grazing estimates by one half. Nevertheless, the community grazing in the water column would still remain one order of magnitude higher than the grazing at the ice-water interface. Even assuming that each species ingestion rate could vary with 5-times more or 5-times less the nominal value, as shown in Table 2, the impact on community grazing is negligible compared to the one order of magnitude difference in grazing between the two habitats (Online resource 1).
Grazing impact (% Chl a), expressed as percentage of phytoplankton standing stock [integrated chlorophyll a biomass at the ice-water interface (0–2 m depth) and in the water column (0–250 m depth)]; a represents total grazing by dominant herbivorous zooplankton species, b represents grazing by larval Antarctic krill
Predation risk
Predation risk by carnivorous zooplankton was expressed as a percentage of the areal abundances of mesozooplankton consumed daily (Table 5), i.e., the dominant herbivorous species selected in this study. At the ice-water interface, total predation risk varied between 0.24 and 2.25% of the mesozooplankton stock, with no apparent spatial pattern. Predation risk was largely dominated by amphipods (mean = 0.50%), followed by gelatinous carnivores. The highest predation risk was found at the most northern station S579_2, due to the presence of ctenophores and a higher contribution of amphipods and siphonophores. At stations where ctenophores were present, their predation pressure accounted for approximately one-third of total predation risk (Table 5). In the water column (0–500 m), total daily predation risk varied between 1.68 and 5.34% of the mesozooplankton stock at all stations, except at one northern station R570_1, where the highest predation risk of almost 20% was inferred, largely due to the contribution of medusae (Fig. 4). Medusae imposed the highest averaged predation risk (2.74%), yet with low frequency of occurrence, followed by chaetognaths (0.88%). Amphipods and ctenophores accounted for only a small part of the total predation risk in the water column. Predation risk in the water column was significantly higher than at the ice-water interface (Wilcoxon rank sum test: W = 4, p < 0.001).
Predation risk by carnivorous zooplankton (% of mesozooplankton), expressed as percentage of mesozooplankton standing stock consumed daily, at the ice-water interface (0–2 m depth) and in the water column (0–500 m depth); mesozooplankton standing stock consists of Antarctic krill larvae and dominant copepods
An integrated comparison of the two habitats, providing an overview on both grazing and predation risk at the same time, indicated that the benefits of the ice-water interface habitat for larval krill outweigh the water column habitat (Fig. 5). Even considering potential errors in our estimates associated with species ingestions rates, such as a reduction in feeding intensity of grazers from summer to winter by 25%, 50% or 75% and of predators by 50%, the advantage of dwelling at the ice-water interface habitat remains strong (Fig. 5).
Biplot of grazing versus predation risk at the ice-water interface layer (0–2 m; SUIT: Surface and Under-Ice Trawl) and the water column (0–500 m; RMT: Rectangular Midwater Trawl); axes are in logarithmic scale. The points represent averaged values estimated at each SUIT and RMT stations using ingestion rates from Table 2. Because winter ingestion rates of grazers were inferred by lowering summer ingestion rates by 50% (showed by the points), horizontal error lines were added to grazing representing a lowering in summer ingestion rates within the range 75–25%. In addition, to represent a potential decrease in winter predation by carnivores, vertical error lines were added within the range 100% (showed by the points using ingestion values from Table 2) to 50%
Discussion
The ice-water interface as a foraging ground
Larval Antarctic krill are dependent upon sea ice to overcome their first winter (Meyer 2012). We hypothesized that the sea-ice habitat hosts fewer competitors and predators of krill larvae compared to the deeper water column, therefore providing an advantage. At the ice-water interface the abundance of larval Antarctic krill was within the same range as the dominant herbivorous species, the ice-associated copepod S. longipes and the pelagic copepods C. propinquus and Ctenocalanus spp (David et al. 2017). In the water column, the areal abundances of pelagic copepods were three orders of magnitude higher than that of krill larvae, indicating that there was more competition for herbivorous food sources. It should be kept in mind that the sampled water column comprised a much larger volume of water (0–500 m) compared to the ice-water interface, which was only integrated over the upper 2 m. Therefore, a higher effort is required in the water column to search for food. Even under the assumption that pelagic copepods were confined within the upper 250 m where phytoplankton resources were available, competition here would have still outweighed the ice-water interface. In the absence of observations on the vertical distribution of zooplankton during our study, we could only speculate upon previously reported winter distributions. Over the sampled depth, krill larvae were, however, observed exclusively in the epipelagic layer either at the sea-ice underside during the daytime (Meyer et al. 2017) or distributed within the upper 20 m at night (Hunt et al. 2014). During winter, C. propinquus is distributed in the upper 200 m of the water column (Schnack-Schiel and Hagen 1995; Pakhomov and Hunt 2014). M. gerlachei and O. similis, the most abundant water column competitors, have a winter distribution spread within the mesopelagic layer (Schnack-Schiel 2001) and were rarely present in our ice-water interface samples, suggesting little or no association with sea-ice habitats. The high number of pelagic copepods in the water column suggests that, at the low chl a concentrations present during our study, their vertical distribution was not entirely driven by autotrophic resources. Therefore, other resources, such as protozoans, small copepods and detritus, must have been available in the water column at that time to sustain the actively feeding populations in winter. For example, experimental studies on O. similis reported preference for motile prey (Sabatini and Kiorboe 1994) or consumption of copepods fecal pellets in the absence of other resources (Gonzales and Smetacek 1994).
At the sea ice underside, juvenile and adult krill, when present, can also compete for food with the krill larvae (Ryabov et al. 2017; Bernard et al. 2019) and even prey upon the krill larvae (Nishino and Kawamura 1994). During our surveys, post-larval krill contributed significantly to the ice-water interface community at only two western stations. Another study from our expedition found that smaller krill larvae were predominant at the ice-water interface (SUIT catches), while larger krill larvae and juveniles were more abundant deeper in the water column (RMT catches) (Schaafsma et al. 2016). A combination of behavioral and physical factors limits the spatial overlapping between development stages (Bergström et al. 1990; Quetin and Ross 1991; Hamner and Hamner 2000). Segregation of krill size ranges or maturity stages are often found in schools or swarms of krill (Quetin and Ross 1991; Watkins 2000; Kawaguchi et al. 2010). Other euphausiids, when present within the same area, can also compete for food resources with larval krill. Since mostly large specimens of the omnivorous T. macrura were collected during our study, we consider them rather predators than competitors of larval krill.
Grazing impact was one to two orders of magnitude lower at the ice-water interface than in the water column. While other species can substantially contribute to grazing, in the present study this was largely due to the high abundance of pelagic copepods. Because of uncertainties in the feeding rates of copepods during winter, we used only half of their lower range values of feeding rates to estimate grazing on phytoplankton stocks. Our water-column values, however, were within the range of values found in the Bellingshausen Sea during spring (Atkinson 1995) and near South Georgia during late summer (Atkinson et al. 1996; Pakhomov et al. 1997). This suggests that the available phytoplankton during late winter/early spring of our sampling period had the capacity to support the active copepod populations, even with inferred ingestion rates from other seasons supposedly higher than winter rates. Our sensitivity analysis on estimated grazing impact using variations in ingestion rates supports the robustness of our conclusions. Nevertheless, our estimates represent only conservative means for synthesising the trophodynamic aspect at the community level. Our approach therefore should be interpreted with caution due to formulated assumptions and it should not replace valuable experimental work. It simply draws the attention to serious gaps in our knowledge on Antarctic zooplankton, which need to be closed in order to evaluate the sea-ice ecosystem values and services.
In addition to phytoplankton, herbivorous zooplankton species at the ice-water interface layer were also feeding on sea-ice biota (Kohlbach et al. 2017, 2018). Stomach contents of krill larvae caught at the ice-water interface during the same expedition were numerically dominated by diatoms and, locally, in terms of biomass by ice-associated copepods, such as S. longipes (Schaafsma et al. 2017). Based on stable isotope measurements of marker fatty acids, the proportion of ingested carbon originating from ice-algae, as opposed to phytoplankton, varied between 20 and 88% depending on sampling location (Kohlbach et al. 2017). By feeding at the ice-water interface, krill larvae would benefit from both lower competition for phytoplankton and from the availability of an important additional food source provided by ice algae and other sea-ice biota (Meyer et al. 2009; Kohlbach et al. 2017; Schaafsma et al. 2017). Estimating the ice-algae standing stock and the grazing rate of ice algae remains difficult. Although spectral irradiance measurements were only possible at three SUIT stations, due to sampling time and limited winter light levels, the little variance between measurements suggests that the amount of chl a was, on average, 40 times higher in the sea ice compared to the upper two meters of the water column. The difficulty in estimating the amount of ice algae available for consumption is, however, that in-ice chl a measurements take the entire sea-ice thickness into account, while the ice algae biomass is concentrated in the bottom 20–30 cm of ice (Meiners et al. 2012). Nevertheless, accounting for ice algae and other sea-ice biota as an available resource at the ice-water interface suggests that feeding in this layer would be considerably more advantageous than estimated by our phytoplankton-based results.
The pack ice region (or region south of 60°S) has been assessed as a food-poor region by a previous study by Meyer et al. (2017), based on stomach contents and growth rates of larval krill. Evidence from this study and previous studies, however, suggest that this might be the case locally, but not true for the entire region. For example, in the center of the sampled area, the larval krill collected were relatively large, had relatively high fatty acid and carbon contents, and had full stomachs (Schaafsma et al. 2016, 2017; Kohlbach et al. 2017). High in-ice chl a concentrations and relatively high grazing impacts by krill and copepods in both depth layers adds to this. Moreover, the estimated grazing rates of larval krill by Meyer et al. (2017) using water column seawater, may have underestimated the importance of ice algae accumulated on sea-ice terrasses, where divers during the same expedition observed larval krill feeding. The growth rates obtained experimentally during the same study were very low reflecting the general winter food scarcity, unlike those observed at marginal ice zone. While Meyer et al. (2017) stated that the pack-ice is a food-poor habitat, their comparison was made in relation to the marginal ice zone and not to the deeper-water column as our study did. Therefore, reduced competition at the ice-water interface compared to the deeper ocean may increase overall sea-ice habitat quality.
The ice-water interface as a shelter against predation
The predation risk by macrozooplankton estimated in the present study was 2–3 times lower at the ice-water interface than in the deeper water column. This was attributed to differences in the community composition and dominant predators within the two habitats. Chaetognaths dominated predation risk in the water column (0.93 ± 0.30), and amphipods at the ice-water interface (0.51 ± 0.33). Our total predation risk in the water column corresponds well with estimates from the Atlantic sector of the Southern Ocean (Pakhomov et al. 1999) and the Indian sector in the vicinity of the Prince Edward Islands (Froneman and Pakhomov 1998). Values by chaetognaths at the ice-water interface during our study corresponded well with predation estimates for meso- and bathypelagic chaetognaths in the Lazarev Sea (Kruse et al. 2010a). The increase in abundance of E. hamata and Sagitta spp. at the ice-water interface stations, where there was also an increase in copepods and krill larvae abundances, suggests that those few chaetognaths foraging within the ice-water interface layer were probably following their potential prey (Froneman and Pakhomov 1998; Kruse et al. 2010a; David et al. 2017).
Previous studies demonstrated that most carnivorous zooplankton are opportunistic predators, consuming the most abundant zooplankton species (Gibbons et al. 1992). For instance, pteropods, gelatinous taxa and even chaetognaths were found in addition to copepods in the guts of the amphipods Themisto gaudichaudii near South Georgia Islands (Pakhomov and Perissinotto 1996; Kruse et al. 2015), and C. lucasii in the Weddell Sea (Lancraft et al. 1991). In the case of chaetognaths’ diet, stomach content analyses have shown the presence of copepods, euphausiids, pteropods and even other chaetognaths (Froneman et al. 1998; Giesecke and González 2012). Hence, predation risk for copepods and krill larvae in our estimates might actually have been lower if other potential prey had been considered. Predation risk by the euphausiid T. macrura remains under debate, since this species is a well-known omnivore, yet has the potential of high predation on mesozooplankton (Pakhomov et al. 1999).
Ctenophores greatly increased the total predation risk within the ice-water interface layer at three stations where they were present. In the water column, other carnivorous gelatinous organisms were responsible for the highest predation risk, such as the medusa Periphylla periphylla and the siphonophore Diphyes antarctica, which collectively accounted for > 50% of the total water column biomass. Medusae, alone, substantially increased the predation impact in the water column at a few stations, with the maximum predation impact of almost 19% at station R570_1. Siphonophores were the only gelatinous group with a relatively constant predation risk over the study area both at the ice-water interface (0.14% ± 0.14) and in the water column (0.19% ± 0.15). In temperate regions, siphonophores have been reported to have the potential to control copepod production, and consequently their grazing impact (Purcell 1981; Mills 1995). Previous studies showed that neither continuous nor indiscriminate feeding could be attributed to gelatinous predators (Purcell 1981, 1991; Gibbons et al. 1992), thus making it difficult to interpret their patterns of occurrence and predation preferences.
In the ice-covered Weddell Sea and Lazarev Sea, mesopelagic fish also feed on copepods and Antarctic krill (Hopkins and Torres 1989; Flores et al. 2008), but the Antarctic krill do not seem to play a major role in their diet (Pakhomov et al. 1996). These fish are known to feed below the epipelagic layer, to avoid visually-cued predators in the upper part of the water column (Robison 2003), where krill larvae were confined during the time of our sampling (Hunt et al. 2014). Although myctophids and paralepidids mesopelagic fish, such as Notolepis coatsi and Bathylagus spp., were present in low numbers in the deeper water column (Pakhomov, unpublished data), they were not included in predation risk because of their absence at the ice-water interface. Furthermore, the sampling gear used was not appropriate to quantitatively estimate the abundance of pelagic fish or other fast swimming nekton. Hence, we did not include fish in our estimate of predation risk.
Large differences in predation risk between the ice-water interface and the water column indicate that krill larvae and other species dwelling at the ice-water interface layer benefit from reduced predation. Divers during the same expedition reported that krill larvae were closely associated with the bottom-ice surface, often taking advantage of structural refuges. Meyer et al. (2009, 2017) proposed that sea ice offers a day habitat for krill larvae during winter, by providing the dual benefit of protection and a feeding ground, thus offering an advantage compared to solely residing in the water column. While the importance of sea-ice as a feeding ground has been proven repeatedly (Daly 1990, Meyer et al. 2009, Jia et al. 2016, Schaafsma et al. 2017, Kohlbach et al. 2017), the advantage of the sea-ice habitat as a shelter from predators has remained largely speculative (Meyer et al. 2017). The results of our study substantiate this hypothesis by demonstrating that the predation risk at the ice-water interface is significantly lower than in the water column.
Conclusions
This study shows that Antarctic krill larvae benefit by dwelling at the ice-water interface compared to the deeper water column. The ice-water interface provided a lower predation risk while offering a phytoplankton standing stock (i.e., in-ice algae) that remained underexploited. While sea ice-derived resources are also important, predator avoidance is an additional, yet poorly assessed benefit of the sea-ice habitat. This dual benefit of sea ice is important to take into account when considering the response of Antarctic krill to projected declines in sea ice. Reduced sea-ice algal production as a result of sea ice decline, may or may not be compensated for by increased water column phytoplankton productivity. However, the shelter provided by the sea ice structures would be significantly reduced or disappear, thus increasing the predation risk on krill larvae.
Data availability
The SUIT dataset is publicly available in the PANGAEA Data Publisher at https://doi.org/10.1594/PANGAEA.909682. The CTD dataset used in the current study is available at https://doi.org/10.1594/PANGAEA.864710. The RMT dataset will be made available at request.
References
Atkinson A (1991) Life cycles of Calanus acutus, CaIanus simillimus and Rhincalanus gigas (Copepoda: Calanoida) within the Scotia Sea. Mar Biol 109:79–89
Atkinson A (1995) Omnivory and feeding selectivity in five copepod species during spring in the Bellingshausen Sea, Antarctica. ICES J Mar Sci 52:385–396
Atkinson A, Shreeve RS, Pakhomov EA, Priddle J, Blight SP, Ward P (1996) Zooplankton response to a phytoplankton bloom near South Georgia, Antarctica. Mar Ecol Prog Ser 14:195–210
Atkinson A, Siegel V, Pakhomov EA, Rothery P (2004) Long-term decline in krill stock and increase in salps within the Southern Ocean. Nature 432:100–103
Atkinson A, Hill SL, Pakhomov EA, Siegel V, Reiss C, Loeb VJ, Steinberg DK, Schmidt K, Tarling GA, Gerrish L, Sailley SF (2019) Krill (Euphausia superba) distribution contracts southward during rapid regional warming. Nat Clim Chang 9:142–147. https://doi.org/10.1038/s41558-018-0370-z
Bathmann U, Makarov R, Spiridonov V, Rohardt G (1993) Winter distribution and overwintering strategies of the Antarctic copepod species Calanoides acutus, Rhincalanus gigas and Calanus propinquus (Crustacea, Calanoida) in the Weddell Sea. Polar Biol 13:333–346. https://doi.org/10.1007/BF00238360
Bergström BI, Hempel G, Marschall HP, North A, Siegel V, Strömberg JO (1990) Spring distribution, size composition and behaviour of krill Euphausia superba in the western Weddell Sea. Polar Rec 26:85–89
Bernard KS, Froneman PW (2003) Mesozooplankton community structure and grazing impact in the Polar Frontal Zone of the south Indian Ocean during austral autumn 2002. Polar Biol 26:268–275. https://doi.org/10.1007/s00300-002-0472-x
Bernard KS, Steinberg DK, Schofield OME (2012) Summertime grazing impact of the dominant macrozooplankton off the Western Antarctic Peninsula. Deep Sea Res Part I 62:111–122
Bernard KS, Gunther LA, Mahaffey SH, Qualls KM, Sugla M, Saenz BT, Cossio AM, Walsh J, Reiss CS (2019) The contribution of ice algae to the winter energy budget of juvenile Antarctic krill in years with contrasting sea ice conditions. ICES J Mar Sci 76:206–216
Castellani G, Schaafsma FL, Arndt S, Lange BA, Peeken I, Ehrlich J, David C, Ricker R, Krumpen T, Hendricks S, Schwegmann S, Flores MH (2020) Large-scale variability of physical and biological sea-ice properties in polar oceans. Front Marine Sci. https://doi.org/10.3389/fmars.2020.00536
Daly KL (1990) Overwintering development, growth, and feeding of larval Euphausia superba in the Antarctic marginal ice zone. Limnol Oceanogr 35:1564–1576
Daly KL, Macaulay MC (1991) Influence of physical and biological mesoscale dynamics on the seasonal distribution and behavior of Euphausia superba in the Antarctic marginal ice zone. Mar Ecol Prog Ser 79:37–66
Daly KL (2004) Overwintering growth and development of larval Euphausia superba: an interannual comparison under varying environmental conditions west of the Antarctic Peninsula. Deep Sea Res Part II 51:2139–2168
David C, Schaafsma FL, van Franeker JA, Lange B, Brandt A, Flores H (2017) Community structure of under-ice fauna in relation to winter sea-ice habitat properties from the Weddell Sea. Polar Biol 40:247–261
Drits A, Pasternak A, Kosobokova K (1993) Feeding, metabolism and body composition of the Antarctic copepod Calanus propinquus Brady with special reference to its life cycle. Polar Biol 13:13–21
Ikeda T (1985) Metabolic rates of epipelagic marine zooplankton as a function of body mass and temperature. Mar Biol 85:1–11
Fetterer F, Knowles K, Meier WN, Savoie M, Windnagel AK (2017) Sea Ice Index, Version 3. Monthly sea ice extent shapefile for September 2013. Boulder, Colorado USA. NSIDC: National Snow and Ice Data Center. https://doi.org/10.7265/N5K072F8. Accessed 20 Sept 2019
Flores H, Van de Putte AP, Siegel V, Pakhomov EA, Van Franeker JA, Meesters EH, Volckaert FA (2008) Distribution, abundance and ecological relevance of pelagic fish in the Lazarev Sea, Southern Ocean. Mar Ecol Prog Ser 367:271–282
Flores H, van Franeker JA, Cisewski B, Leach H, van de Putte A, Meesters EH, Bathmann U, Wolff WJ (2011) Macrofauna under sea ice and in the open surface layer of the Lazarev Sea, Southern Ocean. Deep Sea Res Part II 58:1948–1961
Flores H, van Franeker JA, Siegel V, Haraldsson M, Strass V, Meesters EH, Bathmann U, Wolf WJ (2012) The association of Antarctic krill Euphausia superba with the under-ice habitat. PLoS ONE. https://doi.org/10.1371/journal.pone.0031775
Froneman PW, Pakhomov EA (1998) Trophic importance of the chaetognaths Eukrohnia hamata and Sagitta gazellae in the pelagic system of the Prince Edward Islands (Southern Ocean). Polar Biol 19(4)242–249. https://doi.org/10.1007/s003000050241
Froneman P, Pakhomov EA, Perissinotto R, Meaton V (1998) Feeding and predation impact of two chaetognath species, Eukrohnia hamata and Sagitta gazellae, in the vicinity of Marion Island (Southern Ocean). Mar Biol 131:95–101
Froneman PW, Pakhomov EA, Treasure A (2000a) Trophic importance of the hyperiid amphipod, Themisto gaudichaudi, in the Prince Edward Archipelago (Southern Ocean) ecosystem. Polar Biol 23:429–436
Froneman PW, Pakhomov EA, Perissinotto R, McQuaid CD (2000b) Zooplankton structure and grazing in the Atlantic sector of the Southern Ocean in late austral summer 1993: part 2. Biochemical zonation. Deep Sea Res Part I 47:1687–1702
Gibbons MJ, Stuart V, Verheye HM (1992) Trophic ecology of carnivorous zooplankton in the Benguela. S Afr J Mar Sci 12:421–437
Giesecke R, González HE (2012) Distribution and feeding of chaetognaths in the epipelagic zone of the Lazarev Sea (Antarctica) during austral summer. Polar Biol 35:689–703
Gonzáles HE, Smetacek V (1994) The possible role of the cyclopoid copepod Oithona in retarding vertical flux of zooplankton faecal material. Mar Ecol Progr Ser 113:233–246
Granĺi E, Granéli W, Rabbani MM, Daugbjerg N, Fransz G, Roudy JC, Alder VA (1993) The influence of copepod and krill grazing on the species composition of phytoplankton communities from the Scotia Weddell Sea. Polar Biol 13:201–213
Hagen W, Auel H (2001) Seasonal adaptations and the role of lipids in oceanic zooplankton. Zoology 104:313–326
Hamner WM, Hamner PP (2000) Behavior of Antarctic krill (Euphausia superba): schooling, foraging, and antipredatory behavior. Can J Fish Aquat Sci 57:192–202
Hopkins TL, Torres JJ (1989) Midwater food web in the vicinity of a marginal ice zone in the western Weddell Sea. Deep Sea Res Part A 36:543–560
Hopkin TL, Lancroft TM, Torre II, Donnelly I (1993) Community structure and trophic ecology of zooplankton in the Scotia Sea marginal ice zone in winter (1988). Deep Sea Res Part I 40:81–105
Hunt BPV, Pakhomov EA, Teschke M, King R, Cantzler H, Halbach L, Bose A, Krieger M (2014) Multi-net 24 h stations. In: Meyer B, Auerswald L (eds) The expedition of the research vessel ‘‘Polarstern’’ to the Antarctic in 2013 (ANT-XXIX/7) , vol 674. Reports on Polar and Marine Research, pp 14–18
Jia Z, Swadling KM, Meiners KM, Kawaguchi S, Virtue P (2016) The zooplankton food web under East Antarctic pack ice – a stable isotope study. Deep Sea Res Part II: Top Stud Oceanogr 131189-202. https://doi.org/10.1016/j.dsr2.2015.10.010
Krafft BA, Skaret G, Knutsen T (2015) An Antarctic krill (Euphausia superba) hotspot: population characteristics, abundance and vertical structure explored from a krill fishing vessel. Polar Biol 38:1687–1700
Kawaguchi S, King R, Meijers R, Osborn JE, Swadling KM, Ritz DA, Nicol S (2010) An experimental aquarium for observing the schooling behaviour of Antarctic krill (Euphausia superba). Deep Sea Res Part II 57:683–692
Kirkwood JM (1982) A guide to the Euphausiacea of the Southern Ocean. ANARE Research Notes 1. Australian Antarctic Division, Kingston, p 45
Kruse S, Brey T, Bathmann U (2010a) Role of midwater chaetognaths in Southern Ocean pelagic energy flow. Mar Ecol Prog Ser 416:105–113
Kruse S, Hagen W, Bathmann U (2010b) Feeding ecology and energetics of the Antarctic chaetognaths Eukrohnia hamata, E. bathypelagica and E. bathyantarctica. Mar Biol 157:2289–2302
Kruse S, Pakhomov EA, Hunt BPV, Chikaraishi Y, Ogawa NO, Bathmann U (2015) Uncovering the trophic relationship between Themisto gaudichaudii and Salpa thompsoni in the Antarctic Polar Frontal Zone. Mar Ecol Prog Ser 529:63–74
Kohlbach D, Lange BA, Schaafsma FL, David C, Vortkamp M, Graeve M, Van Franeker JA, Krumpen T, Flores H (2017) Ice algae-produced carbon critical for overwintering of Antarctic krill Euphausia superba. Front Mar Sci 4:310. https://doi.org/10.3389/fmars.2017.00310
Kohlbach D, Graeve M, Lange BA, David C, Schaafsma FL, van Franeker JA, Vortkamp M, Brandt A, Flores H (2018) Dependency of Antarctic zooplankton species on ice algae-produced carbon suggests significant role of ice algae for pelagic ecosystem processes during winter. Glob Chang Biol 24:4667–4681
Lancraft TM, Hopkins TL, Torres JJ, Donnelly J (1991) Oceanic micronektonic/macrozooplanktonic community structure and feeding in ice covered Antarctic waters during the winter (AMERIEZ 1988). Polar Biol 11:157–167
Lange BA, Katlein C, Nicolaus M, Peeken I, Flores H (2016) Sea ice algae chlorophyll a concentrations derived from under-ice spectral radiation profiling platforms. J Geophys Res 121:8511–8534. https://doi.org/10.1002/2016JC011991
Lee DB, Choi KH, Ha HK, Yang EJ, Lee SH, Lee S, Shin HC (2013) Mesozooplankton distribution patterns and grazing impacts of copepods and Euphausia crystallorophias in the Amundsen Sea, West Antarctica, during austral summer. Polar Biol 36:1215–1230
Makorov R, Denys C (1981) Stages of sexual maturity of Euphausia superba. BIOMASS Handb 11:1–13
Marschall HP (1988) The overwintering strategy of Antarctic krill under the pack-ice of the Weddell Sea. Polar Biol 9:129–135
McBride MM, Dalpadado P, Drinkwater KF, Godø OR, Hobday AJ, Hollowed AB, Kristiansen T, Murphy EJ, Ressler PH, Subbey S, Hofmann EE (2014) Krill, climate, and contrasting future scenarios for Arctic and Antarctic fisheries. ICES J Mar Sci 71:1934–1955
Meiners KM, Vancoppenolle M, Thanassekos S, Dieckmann GS, Thomas DN, Tison JL, Arrigo KR, Garrison DL, McMinn A, Lannuzel D, Van der Merwe P (2012) Chlorophyll a in Antarctic sea ice from historical ice core data. Geophys Res Lett 39:L21602. https://doi.org/10.1029/2012GL053478
Metz C, Schnack-Schiel S (1995) Observations on carnivorous feeding in Antarctic calanoid copepods. Mar Ecol Prog Ser 129:71–75
Meyer B, Atkinson A, Stöbing D, Oettl B, Hagen W, Bathmann UV (2002) Feeding and energy budgets of Antarctic krill Euphausia superba at the onset of winter—I. Furcilia III larvae. Limnol Oceanogr 47:943–952
Meyer B, Fuentes V, Guerra C, Schmidt K, Atkinson A, Spahic S, Cisewski B, Freier U, Olariaga A, Bathmanna U (2009) Physiology, growth, and development of larval krill Euphausia superba in autumn and winter in the Lazarev Sea, Antarctica. Limnol Oceanogr 54:1595–1614
Meyer B (2012) The overwintering of Antarctic krill, Euphausia superba, from an ecophysiological perspective. Polar Biol 35:15–37. https://doi.org/10.1007/s00300-011-1120-0
Meyer B, Freier U, Grimm V, Groeneveld J, Hunt BP, Kerwath S, King R, Klaas C, Pakhomov E, Meiners KM, Melbourne-Thomas J, Murphy EJ, Thorpe SE, Stammerjohn S, Wolf-Gladrow D, Auerswald L, Götz A, Halbach L, Jarman S, Kawaguchi S, Krumpen T, Nehrke G, Ricker R, Sumner M, Teschke M, Trebilco R, Yilmaz NI (2017) The winter pack-ice zone provides a sheltered but food-poor habitat for larval Antarctic krill. Nat Ecol Evol 1:1853–1861. https://doi.org/10.1038/s41559-017-0368-3
Mills CE (1995) Medusae, siphonophores, and ctenophores as planktivorous predators in changing global ecosystems. ICES J Mar Sci 52:575–581
Mizdalski E (1988) Weight and length data of zooplankton in the Weddell Sea in austral spring 1986 (ANT V/3). Berichte zur Polarforschung (Reports on Polar Research), p 55
Nishino Y, Kawamura A (1994) Winter gut contents of Antarctic krill (Euphausia superba Dana) collected in the South Georgia area. Proc NIPR Symp Polar Biol 7:82–90
Oresland V (1995) Winter population structure and feeding of the chaetognath Eukrohnia hamata and the copepod Euchaeta antarctica in Gerlache Strait, Antarctic Peninsula. Mar Ecol Prog Ser 119:77–86
Pakhomov EA, Perissinotto R (1996) Trophodynamics of the hyperiid amphipod Themisto gaudichaudi in the South Georgia region during late austral summer. Mar Ecol Prog Ser 134:91–100
Pakhomov EA, Perissinotto R, McQuaid CD (1996) Prey composition and daily rations of myctophid fishes in the Southern Ocean. Mar Ecol Prog Ser 134:1–14
Pakhomov EA, Verheye HM, Atkinson A, Laubscher RK, Taunton-Clark J (1997) Structure and grazing impact of the mesozooplankton community during late summer 1994 near South Georgia, Antarctica. Polar Biol 18:180–192
Pakhomov EA, Perissinotto R, Froneman PW (1999) Predation impact of carnivorous macrozooplankton and micronekton in the Atlantic sector of the Southern Ocean. J Mar Sys 19:47–64
Pakhomov EA, Froneman PW (2004) Zooplankton dynamics in the eastern Atlantic sector of the Southern Ocean during the austral summer 1997/1998—Part 2: Grazing impact. Deep Sea Res Part II 51:2617–2631
Pakhomov EA, Hunt BPV (2014) Pelagic food webs. In: Meyer B, Auerswald L (eds) The expedition of the research vessel “Polarstern” to the Antarctic in 2013 (ANT-XXIX/7), Book 674. Alfred Wegener Institute, Berichte zur Polar-und Meeresforschung, Reports on Polar and Marine Research
Pasternak AF, Schnack-Schiel SB (2001) Seasonal feeding patterns of the dominant Antarctic copepods Calanus propinquus and Calanoides acutus in the Weddell Sea. Polar Biol 24:771–784
Pasternak AF, Schnack-Schiel SB (2007) Feeding of Ctenocalanus citer in the eastern Weddell Sea: low in summer and spring, high in autumn and winter. Polar Biol 30:493–501
Pellichero V, Salee J-B, Schmidtko S, Roquet F, Charrassin J-B (2017) The ocean mixed layer under Southern Ocean sea-ice: seasonal cycle and forcing. J Geophys Res Oceans 122:1608–1633. https://doi.org/10.1002/2016JC011970
Purcell JE (1981) Dietary composition and diel feeding patterns of epipelagic siphonophores. Mar Biol 65:83–90
Purcell JE (1991) A review of cnidarians and ctenophores feeding on competitors in the plankton. In: de Villiers C (ed) Coelenterate biology: recent research on cnidaria and ctenophora. Springer, Dordrecht, pp 335–342
Quetin LB, Ross RM (1991) Behavioral and physiological characteristics of the Antarctic krill, Euphausia superba. Am Zool 31:49–64
Quetin LB, Ross RM, Frazer TK, Haberman KL (1996) Factors affecting distribution and abundance of zooplankton, with an emphasis on Antarctic krill, Euphausia superba. Antarct Res Ser 70:357–371
R-Development-Core-Team (2016) R: a language and environment for statistical computing. R Foundation for Statistical Computing, Vienna. https://www.R-project.org/
Robison BH (2003) What drives the diel vertical migrations of Antarctic midwater fish? J Mar Biol Assoc UK 83:639–642
Ross R, Quetin L (1991) Ecological physiology of larval euphausiids, Euphausia superba (Euphausiacea). Mem Queensl Mus 31:321–333
Ross RM, Quetin LB, Newberger T, Oakes SA (2004) Growth and behavior of larval krill (Euphausia superba) under the ice in late winter 2001 west of the Antarctic Peninsula. Deep Sea Res Part II 51:2169–2184
Ryabov A, de Roos A, Meyer B, Kawaguchi S, Blasius B (2017) Competition-induced starvation drives large-scale population cycles in Antarctic krill. Nat Ecol Evol 1:0177
Sabatini M, Kiørboe T (1994) Egg production, growth and development of the cyclopoid copepod Oithona similis. J Plankton Res 16:1329–1351
Schaafsma FL, David C, Pakhomov EA, Hunt BP, Lange BA, Flores H, van Franeker JA (2016) Size and stage composition of age class 0 Antarctic krill (Euphausia superba) in the ice-water interface layer during winter/early spring. Polar Biol 39:1515–1526. https://doi.org/10.1007/s00300-015-1877-7
Schaafsma FL, Kohlbach D, David C, Lange BA, Graeve M, Flores H, van Franeker JA (2017) Spatio-temporal variability in the winter diet of larval and juvenile Antarctic krill, Euphausia superba, in ice-covered waters. Mar Ecol Prog Ser 580:101–115
Schmidt K, Atkinson A, Pond DW, Ireland LC (2014) Feeding and overwintering of Antarctic krill across its major habitats: the role of sea ice cover, water depth, and phytoplankton abundance. Limnol Oceanogr 59:17–36
Schnack-Schiel SB, Hagen W (1995) Life-cycle strategies of Calanoides acutus, Calanus propinquus, and Metridia gerlachei (Copepoda: Calanoida) in the eastern Weddell Sea, Antarctica. ICES J Mar Sci 52:541–548
Schnack-Schiel SB (2001) Aspects of the study of the life cycles of Antarctic copepods. In: Lopes RM, Reid JW, Rocha CEF (eds) Copepoda: developments in ecology, biology and systematics. Developments in hydrobiology, vol 156. Springer, Dordrecht, pp 9–24
Scolardi KM, Daly KL, Pakhomov EA, Torres JJ (2006) Feeding ecology and metabolism of the Antarctic cydippid ctenophore Callianira antarctica. Mar Ecol Prog Ser 317:111–126
Spreen G, Kaleschke L, Heygster G (2008) Sea ice remote sensing using AMSR-E 89-GHz channels. J Geophys Res 113:C02S03
Swadling KM, Gibson JA, Ritz DA, Nichols PD, Hughes DE (1997) Grazing of phytoplankton by copepods in eastern Antarctic coastal waters. Mar Biol 128:39–48
Torres JJ, Childress JJ (1983) Relationship of oxygen consumption to swimming speed in Euphausia pacifica. I. Effects of temperature and pressure. Mar Biol 74:79–86
Torres JJ, Childress JJ, Quetin LB (1982) A pressure vessel for the simultaneous determination of oxygen consumption and swimming speed in zooplankton. Deep Sea Res 29:631–639
Torres JJ, Aanet AV, Donnelly J, Hopkins TL, Lancraft TM, Ainley DG (1994) Metabolism of Antarctic micronektonic Crustacea as a function of depth of occurrence and season. Mar Ecol Prog Ser 113:207–219
Urban-Rich J, Dagg M, Peterson J (2001) Copepod grazing on phytoplankton in the Pacific sector of the Antarctic Polar Front. Deep Sea Res Part II 48:4223–4246
van Franeker JA, Flores H, van Dorssen M (2009) Surface and Under Ice Trawl (SUIT). In: Flores H (ed) Frozen desert alive—the role of sea ice for pelagic macrofauna and its predators: implications for the Antarctic pack-ice food web. Dissertation, University of Groningen, Groningen, Netherlands
Watkins J (2000) Aggregation and vertical migration. In: Everson I (ed) Krill: biology, ecology and fisheries. Wiley, New York, pp 80–102
Wilcoxon F (1945) Individual comparisons by ranking methods. Biom Bull 1:80–83
Acknowledgements
We thank the captain and crew of RV Polarstern expedition ANT XXIX/7 (Expedition Grant No: AWI-PS81_01 WISKY) for their excellent support with work at sea. We thank Michiel van Dorssen for operational and technical support with the Surface and Under-Ice Trawl (SUIT). We thank Christine Klaas for providing the chlorophyll a data from the water column.
Funding
This work was funded by the Helmholtz Association Young Investigators Group Iceflux: ice-ecosystem carbon flux in polar oceans (VH-NG-800) and PACES (Polar Regions and Coasts in a changing Earth System) program (Topic 1, WP5). SUIT development and SUIT related research by Wageningen Marine Research was supported by the Netherlands Ministry of Agriculture, Nature and Food Quality (LNV; Project WOT-04–009-047.04) and the Netherlands Polar Program (Projects ALW 851.20.011 and ALW 866.13.009). Additional funds were provided by the University of British Columbia and Natural Sciences and Engineering Research Council of Canada (NSERC). During this study Carmen L. David was partly funded from the Canada First Research Excellence Fund, through the Ocean Frontier Institute, and Benjamin A. Lange was partly supported by a Visiting Fellowship from the Natural Science and Engineering Research Council of Canada (NSERC) supported by Fisheries and Oceans Canada International Governance Strategy, in addition to current support from NPI and funding from the Research Council of Norway (CAATEX [280531] and HAVOC [280292]). During this project Brian P.V. Hunt was funded from the European Union’s Seventh Framework Programme for research, technological development and demonstration under Grant Agreement No. 302010—Project ISOZOO.
Author information
Authors and Affiliations
Contributions
CLD, HF and AB designed the research. CLD, FLS and JAF sampled the SUIT stations and processed the samples for species abundance and biomass. EAP and BPVH sampled the RMT stations and processed the samples for species abundance and biomass. HF, BAL and GC processed the SUIT environmental data. CLD wrote the first draft of the manuscript and all authors contributed substantially to revisions.
Corresponding author
Ethics declarations
Conflict of interest
All authors declare no conflict of interest and have approved the final version of the manuscript.
Ethical approval
All international, national, and institutional guidelines for sampling of organisms for this study have been followed.
Additional information
Publisher's Note
Springer Nature remains neutral with regard to jurisdictional claims in published maps and institutional affiliations.
Supplementary Information
Below is the link to the electronic supplementary material.
Rights and permissions
Open Access This article is licensed under a Creative Commons Attribution 4.0 International License, which permits use, sharing, adaptation, distribution and reproduction in any medium or format, as long as you give appropriate credit to the original author(s) and the source, provide a link to the Creative Commons licence, and indicate if changes were made. The images or other third party material in this article are included in the article's Creative Commons licence, unless indicated otherwise in a credit line to the material. If material is not included in the article's Creative Commons licence and your intended use is not permitted by statutory regulation or exceeds the permitted use, you will need to obtain permission directly from the copyright holder. To view a copy of this licence, visit http://creativecommons.org/licenses/by/4.0/.
About this article
Cite this article
David, C.L., Schaafsma, F.L., van Franeker, J.A. et al. Sea-ice habitat minimizes grazing impact and predation risk for larval Antarctic krill. Polar Biol 44, 1175–1193 (2021). https://doi.org/10.1007/s00300-021-02868-7
Received:
Revised:
Accepted:
Published:
Issue Date:
DOI: https://doi.org/10.1007/s00300-021-02868-7